Antioxidant and hepatoprotective effects of Solanum villosum leaf extracts against acetaminophen-induced mouse model of hepatotoxicity
Abstract
Solanum villosum is an indigenous vegetable and ethno-medicine source in Africa. Scientific validation of their medicinal potential is required. The current study aimed to assess the antioxidant and hepatoprotective effects of S. villosum leaf methanol extracts (SVE) against acetaminophen-induced mouse model. The SVE antioxidant activities were evaluated by ferric reducing antioxidant capacity and hydroxyl radical scavenging activities in vitro. Hepatoprotective activities were assessed by automated hematological analysis, chemical analysis and specific biochemical assays of liver homogenates. Mice were orally administered with SVE at 30, 100, 300 and 500 mg/kg body weight. Hepatotoxicity was induced by acetaminophen 300 mg/kg bw intraperitoneally while silymarin 25 mg/kg bw orally was a positive control. The SVE exhibited a clear concentration-dependent antioxidant activity. Subsequently, SVE treatment significantly (P≤0.05) lowered granulocyte count, while there was a significant increase in lymphocyte count in acetaminophen-treated mice. Further, SVE significantly lowered the activities of alanine transaminase, aspartate transaminase, and alkaline phosphatase, but significantly increased γ-glutamyl-transferase activity in treated mice compared to the controls. However, SVE led to insignificant change in total proteins. Additionally, SVE treatment led to a significant rise in superoxide dismutase and glutathione peroxidase activities but, an insignificant change in catalase activity. In conclusion, SVE confers liver protection by oxidative stress prevention, antioxidant enzyme activation, immuno-modulation and hepatic tissue preservation. The results also support indigenous use of S. villosum to protect patients against drug-induced liver damage and enhance immunity. Further, dietary supplements of S. villosum could be an adjunct therapy in acetaminophen-induced hepatotoxicity.
INTRODUCTION
Drug induced liver injury (DILI) remains a public health concern. In the United States and Western countries for instance, DILI is linked to over 50% of acute liver failure incidences, and more than 75% liver transplantations or fatalities [1-3]. Approximately, 70,000 hospitalization cases are reported annually in the US, attributed to acute acetaminophen (ACP) hepatoxicity [4-7]. In sub-Saharan Africa, which suffers acute dearth of current research data on the status of liver diseases especially DILI, and it is estimated that liver diseases-related deaths doubled between 1980 and 2010 [8, 9].
Despite the recent advances in medicine and medical research, a sharp rise in global liver diseases prevalence has been reported, contrary to the narrow range of the available safe drugs used in the management [10, 11]. For instance, N-acetylecysteine remains the main antidote to acute liver failure due to ACP overdose approved for clinical use in the US. This necessitates sustained research for additional therapeutic agents more so for the late presenting cases [7].
The Solanum villosum (Linn.), is one of the species collectively known as ‘African nightshades’ [12-14]. The species grows in the wild but is also cultivated in home gardens and utilized as vegetables, leafy herbs, as well as a source of fruits [15, 16]. Morphologically, the plant grows to an approximate height of 50 cm, has rhombic to ovate leaves and produces red to orange berries upon maturity [6]. Ethno-medicinal uses and reports on scientific evaluation of the medicinal properties of African nightshade extracts have been well published [16-19].
While reports on hepatoprotective effects of S. villosum methanol extracts (SVE) in CCl4-induced liver injury in rats is available [20], an extensive literature review carried out during the current study did not establish authentic information on ameliorative effects of S. villosum on ACP-induced liver injury especially in mouse model. According to McGill [6], mice are a better model for ACP-induced liver injury studies since the toxicity manifestation closely resembles that of humans. Plants remain important source of remedy to various diseases with consumption of specific components or whole plant extracts linked not only to cure, but also reduced toxicity and resistance to many therapeutic drugs [21- 23]. It is on this basis that the current study was designed to evaluate the antioxidant and liver protecting properties of edible SVE in ACP-induced mouse model.
MATERIALS AND METHODS
Chemicals and reagents
Acetaminophen i.v. 1000 mg/100 mL (AdvaCare Pharma, China), silymarin 200 mg (Yangzhou Gami Biochem Co. Ltd., China), ketamine i.v. 100 mg/10 mL (SAGENT Pharmaceuticals, USA) and diazepam i.v. 10 mg/2 mL (Advacare Pharma, China) were purchased from registered dealers in Nairobi Kenya. All other reagents used were analytical grade (AR), from Merck and Sigma-Aldrich, Germany.
Collection of plant samples and processing
Young fresh leaves of S. villosum were randomly collected from Uasin Gishu County (0.5528o, 35.3027o E), Kenya, under the guidance of local farmers in July 2023. The samples were subsequently moistened and then transported in cleaned sacks to Kenyatta University National Therapeutics Research Centre (KUNTRC) laboratories. Part of the samples was taken to National Museum of Kenya, Nairobi Botanical Garden section and authenticated by the institution's indigenous vegetables specialist. After preparation, the specimens were assigned voucher number KUNPRCSV/214/2023, processed and preserved at the Kenyatta University herbarium.
The remaining sample was separately cleaned in running tap water and dried under shade at room temperature for 14 days, with daily combing to ensure uniform aeration. The dry leaves were pulverized into fine powder using an electric blender (ELEKTA® Model No. EFBG-155). The powdered sample was sieved by shaking through a 60 µm mesh sieve, packaged in a 500 g brown paper bag, weighed by a digital balance (Shimadzu Corporation: BL-220H), sealed, labeled, and stored in a refrigerator (Fiocchetti Model No. LAB0500B1TV024) at 4o C.
Extraction of S. villosum leaves
In 2500 cm3 conical flask, 500 g of powdered leaves of S. villosum was separately soaked in analytical reagent grade methanol (Sigma-Aldrich, 99.57%) at a solute-solvent ratio of 1:20. The mixer was placed on an electric shaker to thoroughly mix for 5 min and left to stand on the bench for 48 h. The supernatant was decanted into a clean 2000 mL (Pyrex®) beaker and double filtered first by muslin cloth and then Whatman No.1 qualitative filter paper. The protocol was repeated three times, and the filtrates pooled in a clean 5000 mL conical flask. The filtrate was concentrated by a rotary evaporator at 40oC and then put into pre-weighed clean 100 mL labeled amber sample bottles, covered with perforated aluminum foils, and allowed to evaporate to semisolid extract on the shelf. The extract was then kept in a refrigerator (Fiocchetti M/No. LAB0500B1TV024, Germany) at 4o C. The percentage (%) yields for the extract were computed using following equation as described [24].
Finally, this extraction procedure of S. villosum dried leaves yielded 4.8 % of a dark, green semi-solid extract residue.
In vitro antioxidant activity
Hydroxyl radical scavenging properties
The hydroxyl radicals quenching capacity of MeOH extracts was evaluated following the protocols as described previously [22]. Separately, 500 μL of the extract same to Gallic acid (standard) at dilutions ranging between 15.625 μg/mL to 500 μg/mL, 100 μL of 28.0 mM 2-deoxy-2-ribose dissolved in KH2 PO4 –KOH buffered solution (20.0 mM, pH 7.4), 100μl of EDTA (1.04 mM L−1), 100μL ascorbic acid (1.0 μM), 100 μL FeCl3 (200 mM) and 100 μL hydrogen peroxide (1.0 mM) were freshly blended. The concoctions were incubated at 37 oC for 60 minutes in an electric water bath. Subsequently, 1 % cold 1000 μL each of Thiobarbituric Acid (TBA) and 2.8% Trichloroacetic Acid (TCA) solutions were added to the mixtures before further heating for 15 minutes at 100 oC, till a clear pink color appeared and cooled in cold water. A spectrophotometer (UV/Vis 5100B; Senova Biotech Shanghai, China) was set at 700 nm and zeroed using the blank prepared without the samples or standard solutions added. The optical densities (OD) of both the samples and standard solutions were recorded in triplicates. Subsequently, OD values as a measure of OH radical scavenging activity were plotted against the concentrations in a bar graph.
Ferric reducing antioxidant capacity
The ferric reducing antioxidant capacity (FRAC) of SVE was evaluated in line with the protocol as described [22]. Concisely, 2.5 mL extract solution and Ascorbic acid (standard) in a range of concentrations beginning with 15.625 μg/mL up to 250 μg/mL were placed in a set of cleaned and dried test tubes. Into each, 2.5 mL of 0.2 M phosphate buffer (pH6.6), followed by 2.5 mL of 1% potassium ferricyanide was added and then incubated for 20 minutes at 50oC in a water bath. After cooling, 2.5 mL of 10% trichloroacetic acid was added into each test tube, the mixture vortexed and then centrifuged for 15 min at 3500 rpm. Subsequently, 5 mL of supernatant, 5 mL double distilled water, and 1.0 mL of 0.1% ferric chloride were mixed in a test tube and incubated for 10 min at 25oC (room temperature). A spectrophotometer (UV/Vis 5100B; Senova Biotech Shanghai, China) was set at 700 nm and zeroed using the blank prepared without the samples or standard solutions added. The optical densities (OD) of both the samples and standard solutions were recorded in triplicates. The OD values as a measure of OH radical scavenging activities were plotted against the concentrations in a bar graph for both the standard and the extracts.
Experimental animals
Permission to carry out the research study was issued by the Kenyatta University Ethics Review Committee and the National Commission for Science, Technology, and Innovation (NACOSTI) registration number (NACOSTI/P/23/25686). A total of 35 healthy, female Swiss albino mice aged between 6 to 7 weeks and weighing between 28 and 32 g were procured from the Kenya Medical Research Institute experimental animals’ facility in Nairobi, Kenya.
The mice were randomly separated into seven groups, each consisting of five animals, and housed in 45 cm × 45 cm × 25 cm polypropylene cages. The cage conditions were made habitable using dry, absorbent wood shavings as bedding, replaced after every three days. The mice were subjected to standard laboratory animal keeping conditions, which included 25o C ambient temperature, 12 h of light, and 12 h of darkness cycle. In addition, the study mice had access to certified commercial mouse pellet diet (Unga Ltd., Kenya) and were accorded unlimited access to clean water supply. Subsequently, the mice were allowed time to adapt to their new cages for a period of seven days before the start of the experimentation. The protocol for handling study animals strictly complied with the Organization for Economic Co-operation and Development (OECD) guidelines [25].
The protocol was adopted from a previous study [6]. Mice cages were randomly labeled as mentioned in Table 1. Weights of the mice were taken immediately before the start of the experimentation, once every seven days, and at the end of the treatment period using a digital balance (Shimadzu Corporation: BL-220H, Japan). Control group comprised animals orally administered with 1.5 mL of distilled water once daily (OD) for 21 days. In ACP group, mice were orally administered with 1.5 mL of distilled water OD for 21 days, and on the 18th day, the mice received 300 mg/kg bw ACP intraperitoneally (IP), as a single dose using 27-gauge syringe. In ACP+Sily group (positive control), mice were orally administered with freshly prepared silymarin 25 mg/g bw OD for 21 days and ACP 300 mg/kg bw IP as a single dose on the 18th day. Silymarin was initially dissolved in 3 drops of 1% DMSO and made to the mark using distilled water. In ACP+SVE groups, mice were orally administered with freshly prepared test extracts at 30, 100, 300, and 500 mg/kg bw/d, respectively, for 21 days and APAP 300 mg/kg bw IP as a single dose on the 18th day (Table 1).
The surgical blades, needles and syringes procured were sterile and single use. Each mouse per group was anesthetized using ketamine 100 mg/kg bw and diazepam 5 mg/kg bw IP and euthanized by cervical dislocation on the 22nd day, under the guide of a trained and experienced laboratory technologist. Immediately, the mice were pinned on a dissecting board, the peritoneum cut open and blood drawn from the inferior vena cava with a sterile needle and syringe for each animal. The blood was immediately put into vacutainers containing the anticoagulant ethylenediamine tetraacetic acid (EDTA) and refrigerated at 4oC to be used in a full haemogram test. We strictly adhered to UBC ACC TECH 10a standard operating procedure (SOP) for IP injection in adult mouse and mouse-specific anesthesia guidance by ARC, University of Texas [26]. Subsequently, the mouse liver was excised; blood was blotted out and weighed using a digital balance. Half of the liver was cut off and washed in excess refrigerated normal saline. Liver pieces were homogenized in an ice-cold 0.1 M phosphate buffer solution using an electric tissue homogenizer (KRH-I Lab, KONMIX, China). The homogenates were each centrifuged at 1500 g for 15 min, (Model No. 80-1, Yong Jing, China) the supernatants decanted, and kept refrigerated at 4oC for enzymatic antioxidant assays.
Table 1. Summary of the experimental groups.
Hematological analysis
A 1.5 mL fresh whole blood sample from each mouse was diluted at a ratio of 1:2. Evaluation of hematological parameters followed the principles and procedures as outlined in the autoanalyzer's operating manual (Hematology Analyzer 3960, Dirui Co., LTD., China). Red blood cell count (RBC), hemoglobin (HGB), red cell distribution width (RDW), mean corpuscular hemoglobin (MCH), mean corpuscular hemoglobin concentration (MCHC), and mean cell volume (MCV) were assessed. Leukocytic parameters including monocytes (% MON) count, granulocytes (% GRAN), and lymphocytes (% LYM) counts were also evaluated. Similarly, platelet count (PLT), mean platelet volume (MPV), procalcitonin (PCT), platelet distribution width (PDW), and platelet larger cell ratio (PLCR) were assessed [21].
Liver function analysis
Automated chemical analyzer (Bioassays 240, Dirui Co., LTD., China) was used to evaluate liver function markers according to the manufacturer’s instructions. The markers tested included alanine aminotransferase (ALT), gamma-glutamyl transpeptidase (γ-GGT), aspartate aminotransferase (AST), alkaline phosphatase (ALP) and total protein (TP).
Ex vivo enzymatic antioxidant assays
Superoxide dismutase activity
In superoxide dismutase (SOD) activity assay, superoxide anion (O2-) is generated by autoxidation of hydroxylamine. The O2- formed reduced nitrobluetetrazolium (NBT) forming a formazan dye with absorbance at 560 nm. Addition of SOD preparation clears O2- lowering rate of formazan dye formation. The SOD activity was measured as percentage inhibition of the rate of reduction of NBT as described by [27]. Into a set of cleaned, dried test tubes, 0.2 mL EDTA, 0.4 mL NBT, 1.0 mL Na2CO3 from freshly prepared stock solution and 0.5 mL liver homogenate supernatant from the mice model were added using clean micropipettes and mixed using and vortex shaker (DLAB MX-S50HZ, MedilabTECH, USA). Chemical reactions in the tubes were initiated by the addition of 0.4 mL hydroxylamine hydrochloride solution. 1.5 mL of the mixture was then pipetted into a clean 1.0 cm spectrophotometer cuvette. A spectrophotometer (DLAB SP-UV1000, USA) was blanked using the same mixture contents, excluding 0.4 mL Hydroxylamine hydrochloride solution. Absorbance was read at 560 nm after every 30 s for 3 min and recorded. The procedure was performed in triplicate. SOD activity was computed according to a previous equation [28].
Glutathione peroxidase activity
The glutathione peroxidase (GPx) assay is based on the principle that NADPH is a colored compound that absorbs light maximally at 340 nm and has an extinction coefficient of 6.22 ×103 M-1cm-1, but NADP+ is colorless. GPx activity was calculated as the rate of decrease in absorbance of the reaction mixture. Into a clean dried test tube, 0.1 mL EDTA, 0.1mL Sodium azide, 1.49 mL phosphate buffer (pH 7.4), 0.1 mL GSH, 0.1 NADPH, 0.01 mL H2O2 and 0.1 mL of the liver homogenate supernatant from the mice model were pipetted and mixed in a total volume of 2 mL. The spectrophotometer (DLAB SP-UV1000, USA) was blanked using the same mixture excluding the 0.1ml NADPH solution and absorbance read at 340 nm every 30 s for 3 min. The procedure was performed in triplicate for each sample. Glutathione peroxidase activity was calculated as µmoles of NADPH oxidized in a minute for every mL of the liver homogenate, according to a previous equation [29].
Catalase assay
Catalase (CAT) assay was adopted as described [25]. Briefly, three cleaned test tubes per sample were marked 1, 2, and 3. Into each, 100 µL of the liver homogenate from the mice model, 250 µL phosphate buffer and 200 µL H2O2 were pipetted sequentially. The reactions were stopped at 0.5 s, 30 s, and 60 s through the addition of 1 mL dichromate acetic acid solution into the tubes, followed by boiling at 100oC for 10 min. The tube contents were allowed to cool to room temperature and absorbance was read at 620 nm using a spectrophotometer (DLAB SP-UV1000, USA). A blank was prepared by the same procedure except for the sample replaced by a similar volume of distilled water. The assays were performed in triplicate. Catalase activity was calculated using a previous equation [30]. Catalase activity was expressed as µmoles of H2O2 decomposed per min/mL of homogenate.
Statistical analysis
The data obtained from in vitro antioxidant assays, evaluation of hematological changes, liver function test assays, and ex vivo enzymatic analyses were keyed into Microsoft Excel (2010) spreadsheet, categorized, transferred to and analyzed using GraphPad prism version 8.0.2(263). Prior to analysis, data was checked for normalcy by Shapiro-Wilk test at P≤0.05. Data analysis was performed by one-way ANOVA, followed by Tukey's post hoc multiple comparison test at α = 95% and P ≤ 0.05. The outputs were expressed in tables and figures.
RESULTS
Effect of SVE on hydroxyl radical scavenging activity
The hydroxyl radical scavenging activities of SVE increased gradually with an increase in extract concentrations similar to gallic acid, the standard. However, at all dosages, gallic acid had statistically significantly (P≤0.05) higher OH radical scavenging activity compared to SVE (Figure 1).
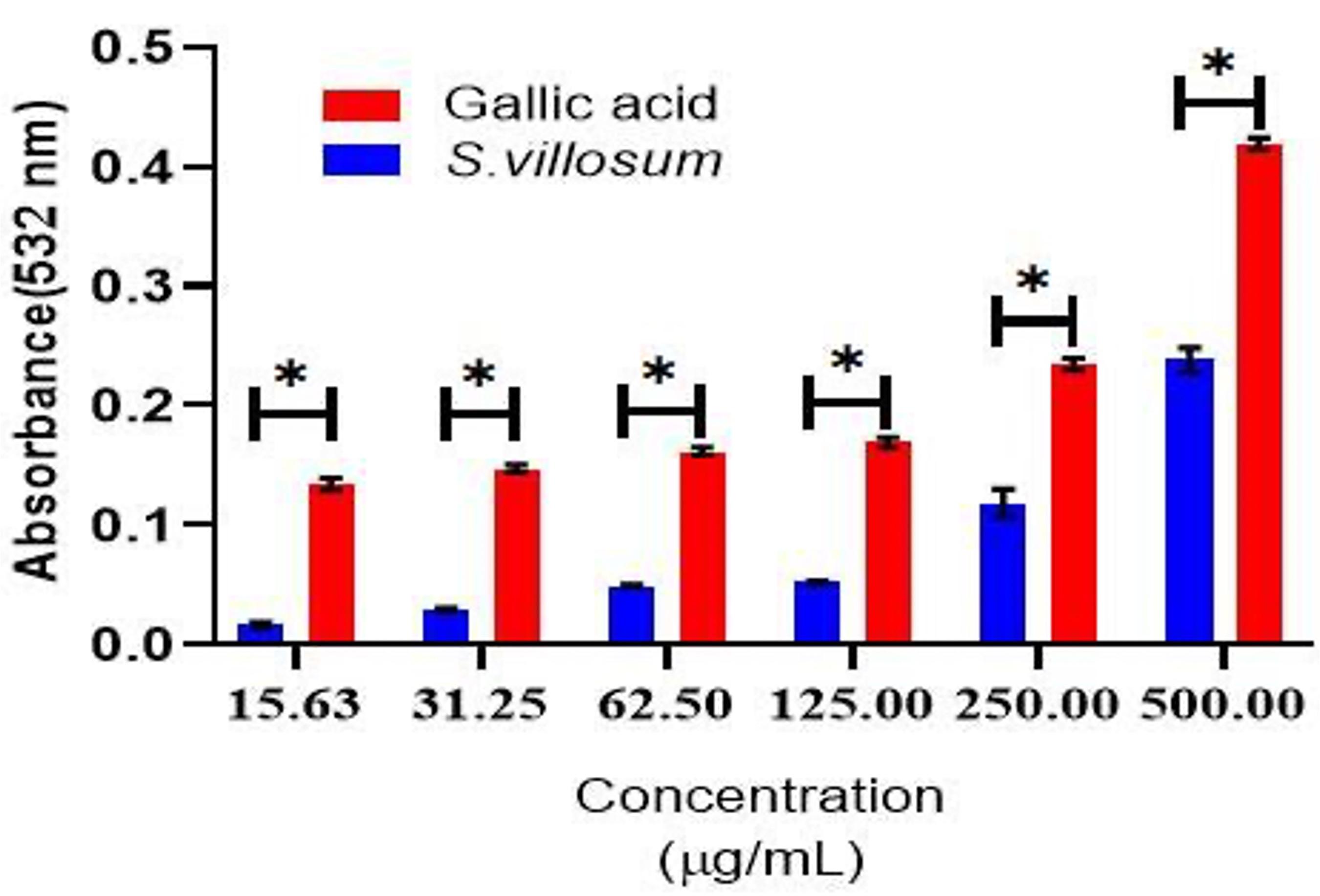
Effect of SVE on ferric reducing antioxidant capacity
Likewise, FRAC test results showed that SVE had obvious antioxidant activity as observed by the concentration dependent increase in absorbance. However, the crude extract activities were significantly lower than those of ascorbic acid, except at the 15.63 μg/mL level (Figure 2).
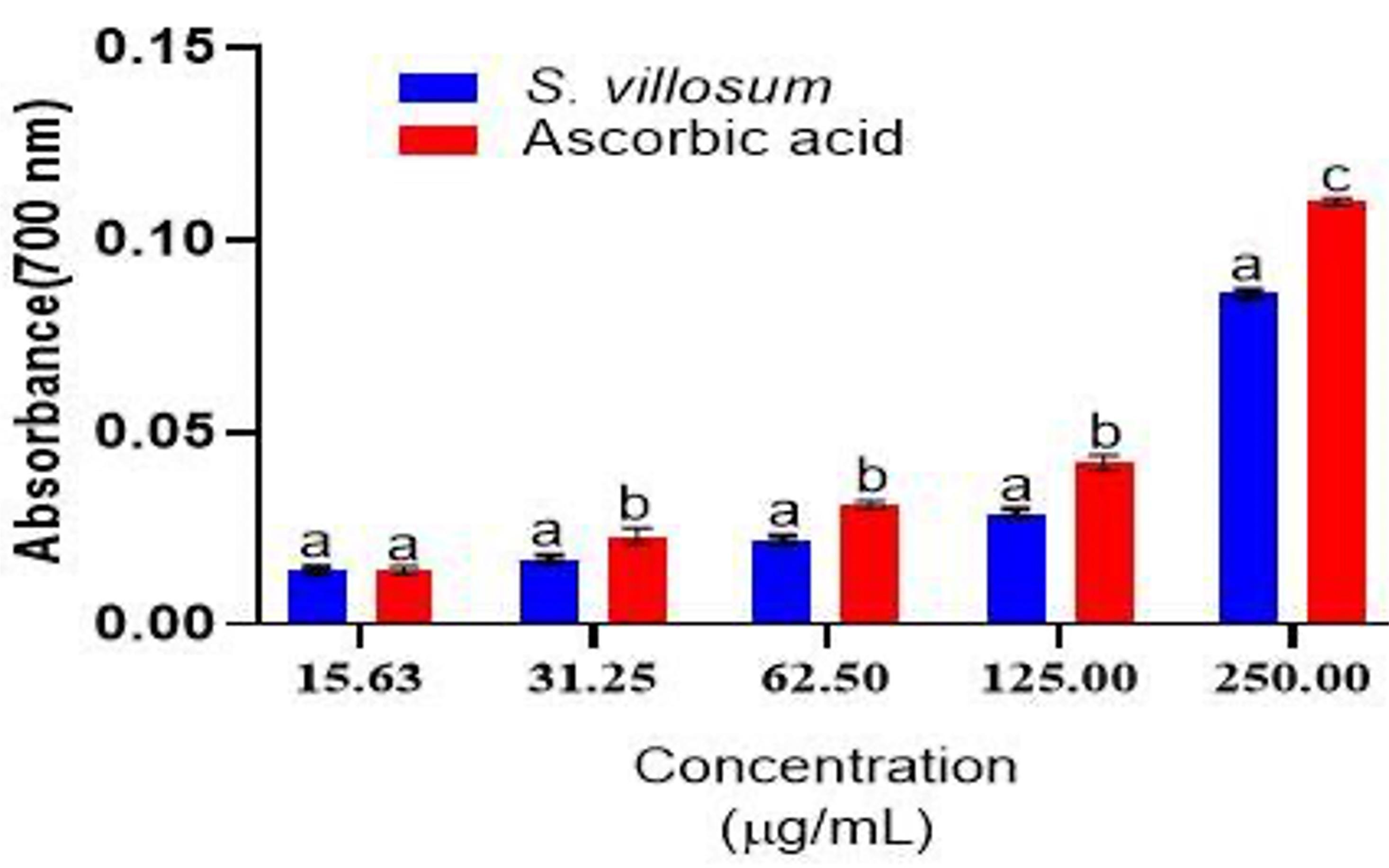
Effect of SVE on red blood cells, platelets and related parameter profiles
The administration of ACP at 300 mg/kg bw intraperitoneally did not lead to statistically significant changes in RBC and related parameter profiles in all the treatment groups (Supplementary Table 1). However, there was a statistically significant drop in total PLT and PCT, respectively in all groups of mice treated with ACP 300 mg/kg bw compared to the normal control group (Supplementary Table 2).
Effects of SVE on leukocytic parameter profiles
Treatment with ACP at 300 mg/kg bw led to an overall statistically significant (P≤0.05) drop in the white blood cells (WBC) as observed between the normal control group compared to all treated groups. However, a statistically significant (P≤0.05) increase in WBC was observed in the groups treated with SVE at different dosages. It was noteworthy that the extract activity was non-dose dependent (Figure 3A). The study results also showed that SVE at all dosages led to statistically significant (P≤0.05) increments in lymphocyte population (% LYM) in mice induced with ACP 300 mg/kg bw, compared to normal and the negative control. Nonetheless, the observed activities were non-dose dependent (Figure 3B). Additionally, it was observed that administration of ACP significantly increased the granulocyte counts. However, in group treated with SVE, granulocyte counts were significantly reduced in a dose-dependent manner. The extract activities at 100, 300 and 500 mg/kg bw were comparable to the positive control (Figure 3C).
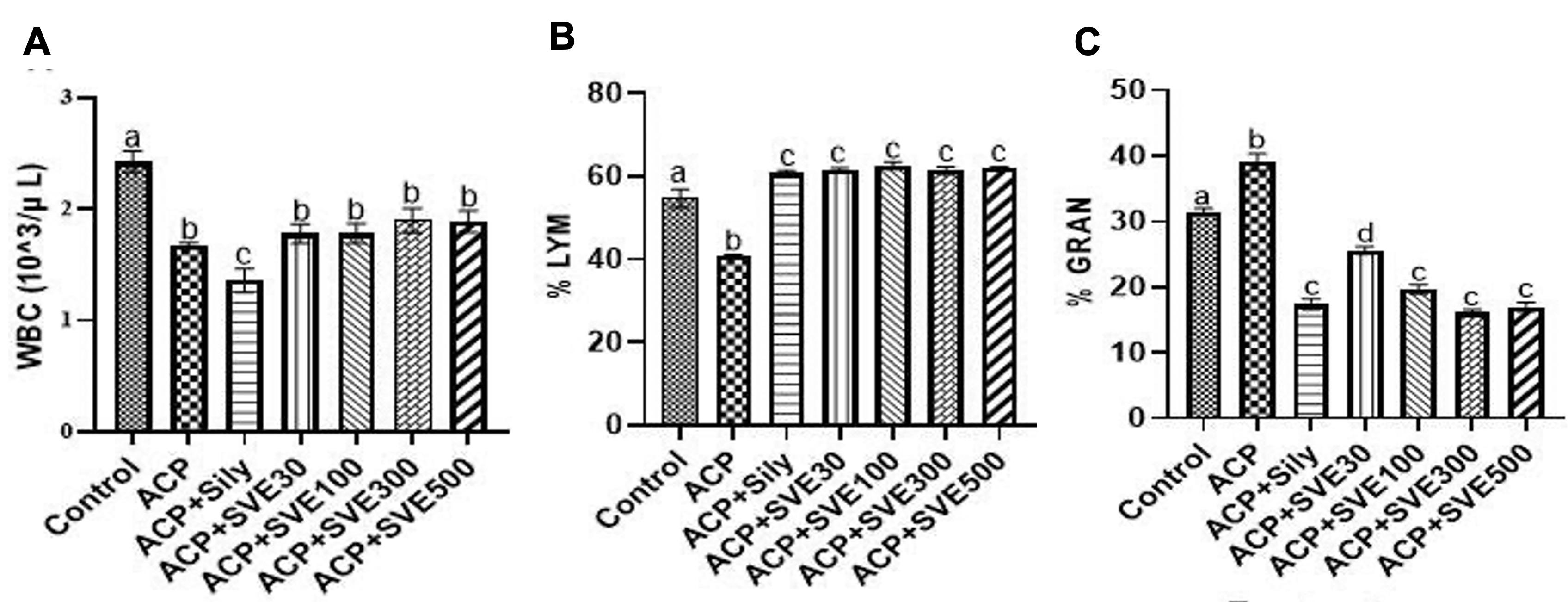
Effect of SVE on liver function markers
The study result revealed that ACP 300 mg/kg bw as a single dose administration led to hepatocytes damage in mice as demonstrated by the statistically significant elevation in ALP, ALT, AST and γ-GGT. There was a significant drop in total protein. However, treatment with SVE resulted in significant declines in the serum concentrations of ALP, ALT, AST, and γ-GGT respectively. The extract activities were dose dependent. At 300 and 500 mg/kg bw, SVE effects on ALT and AST were comparable to that of the standard drug silymarin 25 mg/kg bw. Nonetheless, the extract did not significantly prevent the drop in total protein across all the treated groups (Table 2).
Table 2. Effect of extract on liver function markers in ACP-induced mice model.
Effect of SVE on antioxidants in the liver
Liver damage by ACP 300 mg/kg bw lead to significantly (P≤0.05) low SOD activity compared to the normal control. However, in mice treated with SVE extracts, SOD activities were normal with insignificant variations in a dose-dependent manner (Figure 4A). Similarly, it was observed that ACP 300 mg/kg bw i.p. injection led to significantly (P≤0.05) low GPx activity in the negative control group compared to the normal control group. However, in mice treated with SVE, GPx activity increased in a dose-dependent manner. It was observed that SVE lead to higher GPx activity at all dosages compared to the positive control, silymarin 25 mg/kg bw (Figure 4B). Nevertheless, there was no statistically significant change in catalase activity between the control groups and all the groups treated with SVE extract (Figure 4C).
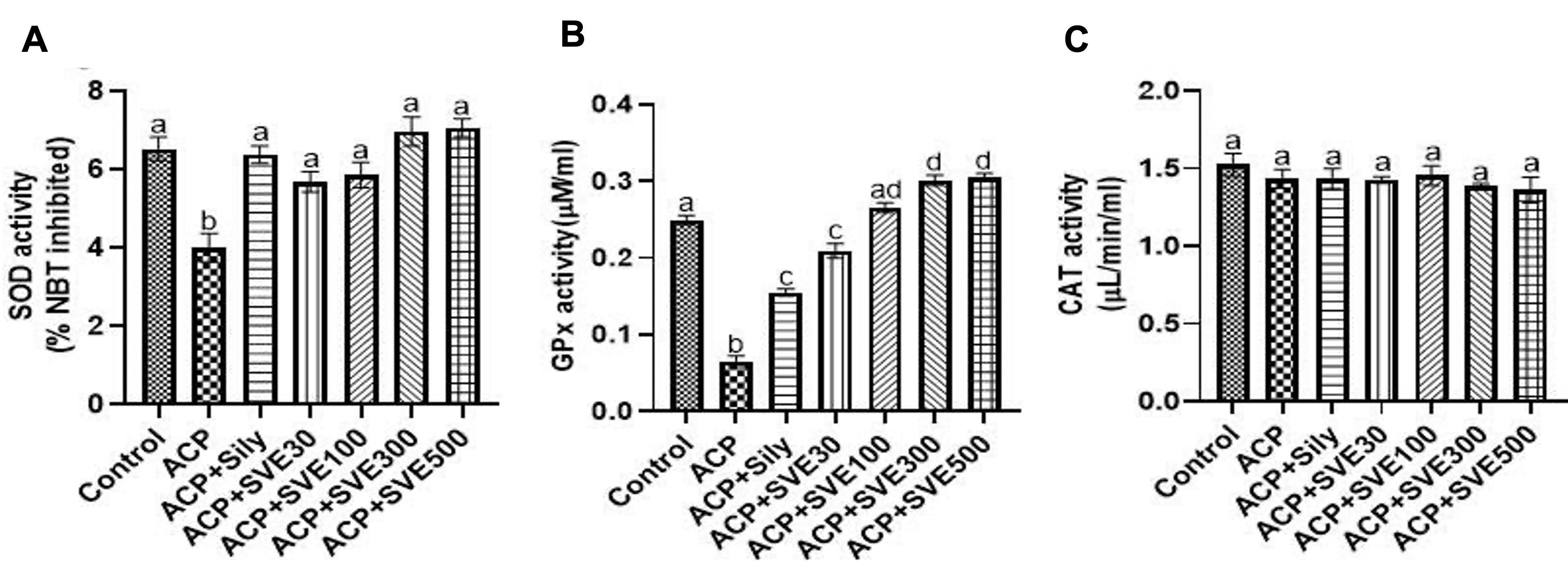
DISCUSSION
ACP is a globally used, generally safe analgesic and antipyretic drug when taken in the right dosage. Nonetheless, ACP hepatotoxicity is widely documented as the leading cause of acute liver failure in the global west. It contributes to significant hospitalization cases yearly and hence a public health concern [4, 32]. Factors contributing to a high probability of ACP-induced hepatotoxicity occurrence include ease of access, frequent oblivious use of ACP combination products, as well as incidences of suicide attempts [7]. ACP remains the drug of choice in an experimental model for DILI of clinical importance since the mechanism of toxicity is fairly well understood [6, 7]. Principally, at the normal dose, up to 90% of ACP administered is glucuronidated or sulfated and then, eliminated through the kidneys. The remaining percentage are degraded by the cytochrome P450 enzymes, majorly the Cyp2E1 and Cyp1A2 isoforms into the very reactive metabolite N-acetyl-p-benzoquinone imine (NAPQI). Under normal circumstances, the low concentration of NAPQI produced is actively conjugated with glutathione and eliminated in bile leading to negligible liver toxicity [31].
Administration of greater than ACP 7.5 g as a single dose oversaturates the sulfation pathway and high concentration of NAPQI if formed. NAPQI rapidly attaches to reduced glutathione (GSH) via covalent linkage and depletes GSH in hepatocytes. The reactive metabolite also bonds with sulfhydryl groups of various cellular proteins forming APAP protein adducts. The formation of mitochondrial protein adducts initiates mitochondrial damage through heightened production of reactive oxygen species (ROS), mainly superoxide and peroxy-nitrites [2, 4, 5, 7]. The peroxy-nitrite radical has the potential to further change the conformation of other protein structures, by nitration of tyrosine residues and all contributing to hepatocytes necrosis [4; 31; 32].
In the current study, the in vitro FRAC and hydroxyl radical scavenging activity assays of SVE showed marked dose-dependent antioxidant activities. Antioxidants quench free radicals, reactive oxygen species (ROS) and reactive nitrogen species (RNS), whose unchecked build-up in the body cells results in oxidative stress [22; 24; 33; 34]. The use of a polar solvent such as MeOH in extraction obtains polar secondary metabolites from plant tissues, with documented antioxidant activities. For instance, the analysis of the metabolite profiles of SVE [35] revealed the presence of saponins, steroidal glycoalkaloids and polyphenols which they linked to various bioactivities. Similarly, Ahmed [36] extracted and compared the composition and antioxidant activities of C. caudutum using MeOH, H2O and C6H14. They reported that MeOH extracts had the highest antioxidant activity of the three solvents. In the present study, we attributed the low GPx and SOD activities observed in the negative control group to the toxicity effects of NAPQI. Nonetheless, the increased activities of GPx and SOD were associated with the antioxidant and enzyme inductive properties of S. villosum MeOH extract. Biochemically, GPx catalyzes the breakdown of the noxious H2O2 into safe H2O. Studies have shown that overexpression of specifically GPx1 isoform confers protection against hepatic oxidative damage [37]. Similarly, the role of superoxide dismutase in mediating hepatotoxicity in mice has been reported. Specifically, the copper-zinc-containing superoxide dismutase (CuZnSOD) isoform is strategically located in the inter-membrane space of mitochondria. Here, it confers enhanced mitochondrial protection against superoxide radicals (O2-). It also acts to barricade superoxide radicals from escaping out of the mitochondria [6]. However, contrary to the finding by [35] in isoniazid/rifampicin-induced toxicity in rats; there were no significant changes in catalase activity. This was attributed to the knowledge that catalase activity is largely confined to the peroxisomes, an organelle that lacks the target proteins for NAPQI [6]. Silymarin, the positive control drug in the current study, exerts liver protective activity by reducing malondialdehyde concentration and induction of endogenous antioxidant enzyme systems including SOD, CAT, and GPx [27]. As reported by other studies, ACP-induced hepatotoxicity in mice manifests as an intense inflammatory reaction [7, 31, 32]. This was confirmed by the significant increase in granulocyte populations in the negative control group. However, the contradictory statistically insignificant change in the monocytes was linked to a possible stimulation of the liver myeloid precursor cells by ACP but not the bone marrow cells [38]. Lymphocytes (B and T cells) belong to the lymphoid lineage of the leucocytes. Their commitment to differentiating hematopoietic stem cells (HSC) is subject to influence by cytokines kinases and trypsin kinases. The interleukins (IL) involved in differentiation of HSC into lymphoid precursors include IL-1, IL-2, IL-3, IL-6 and IL-7 [39]. Plant phenolics are known to initiate immune response by influencing factors that control proliferation, differentiation and maturity of white blood cells. For instance, silymarin enhances the release of interferon-gamma (IFN-γ), IL-4 and IL-10 [27; 40]. The observed statistically significant drop in PLTs and PCT respectively could be attributed to inhibition of megakaryocyte activity by ACP. Inadequate platelet production or malfunctions in the regulation of thrombopoiesis could similarly contribute to diminished platelet numbers [41; 42]. The intense release of APL, AST, ALT and γ-GGT is often correlated with the integrity status of hepatocytes [7; 31; 43]. The γ-GGT is important in degradation and synthesis of glutathione as well as detoxification of drugs and xenobiotics. Biochemically, AST catalyzes the translocation of α-amino group from L-aspartate to α-ketoglutarate, to produce oxaloacetate and L-glutamate. On the other hand, ALT catalyzes the transfer of α-amino group (NH2) from L-alanine to α-ketoglutarate, to form pyruvate and L-glutamate respectively [44]. Elevated plasma concentration of these two enzymes above the maximum normal level (ULN) is clinically associated with DILI, hepatitis, cirrhosis and mononucleosis [4]. In a related study, Abdel‑Hamid [20] reported the hepatoprotective and antifibrotic activities of SVE in rat model induced by administration of CCl4. They explained that the species extracts acted by antioxidant activity as well as regulation of the expression of fibrogenic mediators.
The researchers concluded that hepatoprotective activities of SVE are comparable to those of silymarin. They attributed the resultant activities to secondary metabolites in the plants extract. Similarly, protective effects of S. nigrum methanol extract against isoniazid and rifampicin-induced hepatotoxicity in rats were evaluated [35]. The result showed ameliorative activities of the extract against the hepatotoxicity effects of the regimen similar to our observations in the present study.
CONCLUSIONS
Oral administration of SVE to female mice protected them from ACP-induced hepatotoxicity. The activity of the extract is dose dependent. Further, the extract was demonstrated to act by free radical scavenging, anti-inflammatory, and induction of various enzymatic antioxidant activities (Figure 5).
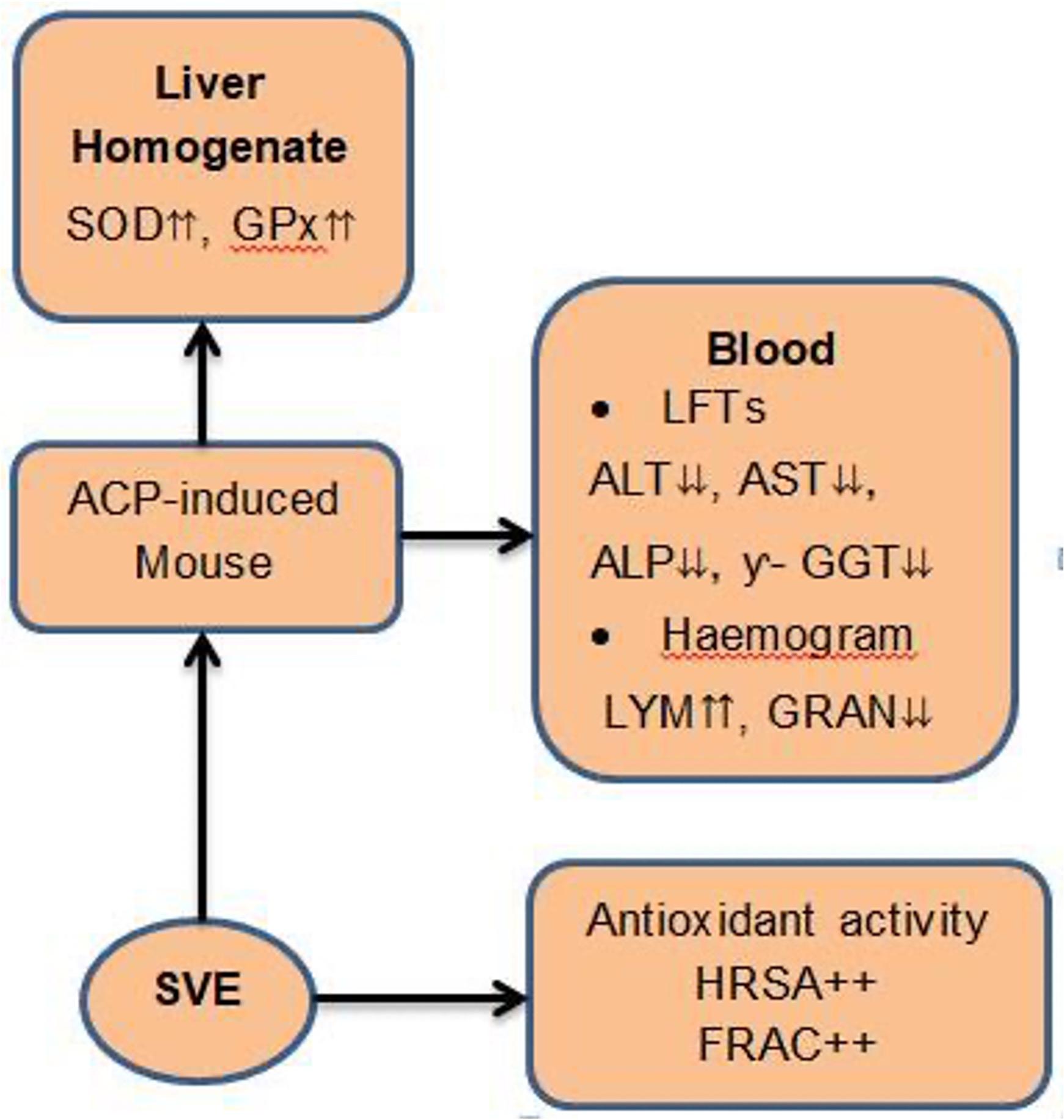
Owing to the comparable manifestation of ACP toxicity in mice and humans [6, 45], it is concluded that dietary consumption of S. villosum may also confer liver protection against ACP-induced hepatotoxicity in humans. Therefore, upon further evaluation, edible S. villosum leaves may be processed into a novel product that can be used as an adjunct in the management of ACP-induced hepatotoxicity.
ACKNOWLEDGEMENTS
The research study was carried out in Biochemistry laboratories and animal house in the Department of Biochemistry, Microbiology and Biotechnology, Kenyatta University, Kenya. The authors received a partial grant from the National Research Fund [NRF/PhD/02/79] to purchase laboratory consumables. The authors greatly appreciate the logistical assistance we were accorded from the Department of Biochemistry, Microbiology and Biotechnology. Similarly, the authors appreciate Uzima University for their publication support.
AUTHOR CONTRIBUTIONS
AKM and MPN contributed to the design and proofreading of the paper. KOO designed, executed the experiment and analyzed the data under the supervision of AKM and MPN. KOO drafted the manuscript. All authors have approved the final version.
CONFLICTS OF INTEREST
There is no conflict of interest among the authors.
SUPPLEMENTARY MATERIALS
Supplementary Table 1. Effects of SVE on RBCs and related parameters and Supplementary Table 2. Effect of SVE on platelets and related parameters (Supplementary materials).
References
- [1]Kaplowitz, N. Drug-Induced Liver Injury. Clinical Infectious Diseases. 2004; 38, S44-S48.
- [2]Chen M, Suzuki A, et al. Drug-induced liver injury: interactions between drug properties and host factors. Journal of hepatology. 2015; 63: 503–514.
- [3]Kullak-Ublick GA, Andrade RJ, et al. Drug-induced liver injury: Recent advances in diagnosis and risk assessment. Gut. 2017; 66: 1154–1164.
- [4]Laura JP, Mayeux PR, et al. Acetaminophen-induced hepatotoxicity. Drug metabolism and disposition. 2003; 31: 1499–1506.
- [5]Bajt ML, Knight TR, et al. Acetaminophen-induced oxidant stress and cell injury in cultured mouse hepatocytes: Protection by N-acetyl cycteine. Toxicological Sciences. 2004; 80:343–349.
- [6]McGill MR. Acetaminophen-induced Hepatoxicity. Nat Rev Cancer. 2011;12:237–251.
- [7]Ramachandran A, Jaeschke H. Acetaminophen toxicity: Novel insights into mechanisms and future perspectives. Gene Expr 2018;18:19–30.
- [8]Vento S, Dzudzor B, et al. Liver cirrhosis in sub-Saharan Africa: neglected, yet important. The Lancet Global Health. 2018; 6: e1060–e1061.
- [9]Otedo A, Simbiri KO, et al. Risk Factors for Liver Cancer in HIV Endemic Areas of Western Kenya. Journal of Infectious Agents and Cancer. 2018; 13:1-9.
- [10]Rao AS, Ahmed MF, and Ibrahim M. Hepatoprotective Activity of Melia azedarach Leaf Extract against Simvastatin Induced Hepatotoxicity in Rats. Journal of Applied Pharmaceutical Science. 2012; 7: 144-148.
- [11]Shirani M, Raeisi R, Heidari-Soureshjani, S, et al. A Review for Discovering Hepatoprotective Herbal Drugs with Least Side Effects on Kidney. Journal of Nephropharmacology. 2017; 6: 38-48.
- [12]Poczai P, Hyvönen J, et al. On The Origin of Solanum nigrum: Can Networks Help? Molecular Biology Reports. 2011; 38:1171-1185.
- [13]Sundari SG, Rekha S, et al. Phytochemical Evaluation of Three Species of Solanum L. International Journal of Research in Ayurveda and Pharmacy (IJRAP).2013; 4:420-425.
- [14]Klocke E, Matasyoh LG, et al. Biotechnological tools for improvement of black nightshade (Solanum nigrum L. complex), valuable medicinal and vegetable plants in Kenya. 6th Int.Symp Breed Res Med Aromat Plants (BREEDMAP 6). 2016;453:22–56.
- [15]Gido EO, Ayuya OI, et al. Consumption intensity of leafy African indigenous vegetables: towards enhancing nutritional security in rural and urban dwellers in Kenya. Agric Food Econ. 2017;5:0–16.
- [16]Kimiywe J, Waudo J, et al. Utilization and Medicinal Value of Indigenous Leafy Vegetables Consumed in Urban and Peri-Urban Nairobi. African J. Food, Agric. Nutr. Dev. 2007; 701–15.
- [17]Ojiewo CO, Mwai GN, Abukutsa-Onyango MO, Agong SG, Nono-Womdim R. Exploiting the genetic diversity of vegetable African nightshades. Bioremediation, Biodiversity and bioavailability. 2013;7(1):6-13.
- [18]Atanu FO, Ebiloma UG, et al. A review of the pharmacological aspects of Solanum nigrum Linn. Biotechnol Mol Biol Rev. 2011; 6:1–007.
- [19]Krithika R, Verma RJ. Solanum nigrum confers protection against CCl4-induced experimental hepatotoxicity by increasing hepatic protein synthesis and regulation of energy metabolism. Clinical Phytoscience. 2019; 5: 1–8.
- [20]Abdel‑Hamid AE, Dine RS, et al. Metabolic profiling of Solanum villosum mill. sub sp. miniatum (bernh. ex willd.): Hepatoprotective and antifibrotic activity in a rat model of liver fibrosis. Pharmacology Magazine. 2019; 15:659-70.
- [21]Osano KO, Nyamai DW, et al. Evaluation of In Vivo Toxicity of Dichloromethane: Methanolic Leaf Extracts of Prosopis juliflora in Female Wistar Albino Rats. J. Drug Metab Toxicol. 2015; 01:1–11.
- [22]Nyalo P, Omwenga G, et al. Quantitative Phytochemical Profile and In Vitro Antioxidant Properties of Ethyl Acetate Extracts of Xerophyta spekei (Baker) and Grewia tembensis ( Fresen ). JABIM. 2023; 28: 1-15.
- [23]Waziri P, Auta R, Imam MU, et al. In Vivo Anti-Hepatocellular Carcinoma Effects of the Chloroform Root Extract of Clausena excavata Burm. Journal of Evidence-Based Integrative Medicine. 2024; 9:1-8.
- [24]Moriasi GA, Ireri AM, et al. In vivo anti-inflammatory, anti-nociceptive, and in vitro antioxidant efficacy, and acute oral toxicity effects of the aqueous and methanolic stem bark extracts of Lonchocarpus eriocalyx (Harms.). Heliyon, 2021;7.
- [25]Saleem U, Amin S, et al. Acute oral toxicity evaluation of aqueous ethanolic extract of Saccharum munja (Roxb.) roots in Swiss albino mice as per OECD 425 TG. Toxicology Reports, 2017; 4: 580–585.
- [26]Cicero L, Fazzotta S, et al. Anesthesia protocols in laboratory animals used for scientific Purposes. Acta Biomedica. 2018; 89: 337–342.
- [27]Al-Enazi MM. Protective effects of combined therapy of rutin with silymarin on experimentally-induced diabetic neuropathy in rats. Pharmacology & app; Pharmacy. 2014; 05: pp. 876–889.
- [28]Zhang C, Bruins ME, et al. A new formula to calculate activity of superoxide dismutase in indirect assays. Analytical Biochemistry. 2016; 503: 65–67.
- [29]Weydert C, Cullen J. Measurement of superoxide dismutase, catalase, and glutathione peroxidase in cultured cells and tissue. Cell Moleculat Biology. 2011; 5:51–66.
- [30]Sampson R, Abraham S. Impact of four oral antiretroviral drugs on human erythrocyte catalase activity ( in vitro). East African Scholars Journal of Medical Sciences.2019; 4421: 165–168.
- [31]Yuan L, and Kaplowitz N. Mechanisms of drug-induced liver injury. Clinics in Liver Disease. 2013; 4: 507–518.
- [32]Yan M, Huo Y, Yin S, et al. Mechanisms of acetaminophen-induced liver injury and its implications for therapeutic interventions. Redox Biology. 2018; 17: 274–283.
- [33]Srinivasa RA, Ahmed MF, et al. Hepatoprotective activity of melia azedarach leaf extract against simvastatin induced hepatotoxicity in rats. Journal of Applied Pharmaceutical Science. 2012; 2:144–148.
- [34]Ighodaro OM, Akinloye OA, et al. First line defence antioxidants-superoxide dismutase (SOD), catalase (CAT) and glutathione peroxidase (GPX): Their fundamental role in the entire antioxidant defence grid. Alexandria Journal of Medicine. 2018;54: 287–293.
- [35]Fayed AM, Salama A A A, et al. Protective Effects of Solanium nigrum Methanolic Extract Against Isoniazid / Rifampicin Induced Hepatotoxicity In Rats. J. Appl. Vet. Sci. 2019; 4:52–61.
- [36]Ahmed D, Khan MM, et al. Comparative analysis of phenolics, flavonoids, and antioxidant and antibacterial potential of methanolic, hexanic and aqueous extracts from Adiantum caudatum leaves. Antioxidants. 2015; 4:394–409.
- [37]Rahman I, Kode A, Biswas SK, et al. Assay for quantitative determination of glutathione and glutathione disulfide levels using enzymatic recycling method. Nature Protocols. 2007; 1:3159–3165.
- [38]Ginhoux F, Jung S, et al. Monocytes and macrophages: Developmental pathways and tissue homeostasis. Nature Reviews Immunology. 2014; 14: 392–404.
- [39]Marti LC, Bacal NS, et al. Lymphoid Hematopoiesis and Lymphocytes Differentiation and Maturation. Lymphocyte Updates – Cancer, Autoimmunity and Infection. 2017; 23-45.
- [40]Comelli MC, Mengs U. Toward the definition of the mechanism of action of silymarin: Activities related to cellular protection from toxic damage induced by chemotherapy. Integrative Cancer Therapies. 2007; 6:120–129.
- [41]Vagdatli E, Gounari E, et al. Platelet distribution width: A simple, practical and specific marker of activation of coagulation. Hippokratia. 2010; 14: 28–32.
- [42]Budak YU, Polat M, et al. The use of platelet indices, plateletcrit, mean platelet volume and platelet distribution width in emergency non-traumatic abdominal surgery: systematic review. 2016; 3:178–193.
- [43]Delgado-Montemayor C, Cordero-Pérez P, et al. Models of hepatoprotective activity assessment. Medicina Universitaria. 2015; 17: 222–228.
- [44]Arika WM, Nyamai DW, Osano KO, et al. Biochemical Markers of In Vivo Hepatotoxicity. Journal of Clinical. Toxicol. 2016; 6: 297.Doi:10.4172/2161-0495.1000297.
- [45]Suh JI. Drug-induced liver injury. Yeungnam University Journal of Medicine. 2020; 33: 2.