Effect of aqueous and ethanol extracts of Cucurbita maxima seed on fasting blood glucose in alloxan-induced rats
Abstract
Diabetes is a chronic disorder marked by hyperglycemia due to insulin resistance and insufficient insulin action, or both. Synthetic antidiabetic drugs available on the market are associated with severe effects, necessitating the need for alternative antidiabetic drugs. This investigation aimed to determine the antidiabetic potential and phytochemical profiles of aqueous and ethanol seed extracts of Cucurbita maxima. The seeds of C. maxima were airdried, milled, and extracted using distilled water and ethanol. The antidiabetic effect of the extracts was performed using Wistar rats weighing 160-180 g. Thirty-five rats were assigned to 7 groups: non-diabetic (control), diabetic, glibenclamide-treated, and aqueous or ethanol extract-treated (200 and 400 mg/kg body weight). Alloxan monohydrate was used to induce experimental diabetes. The treatments were orally administered for 28 days. Blood glucose levels and histopathological analysis were performed. The phytochemical screening was conducted utilizing liquid chromatography-mass spectrometry (LC-MS). In diabetic rats, the effects of the two extracts revealed a substantial reduction in levels of fasting blood glucose in a dose-dependent manner, suggesting an antidiabetic effect. The histopathological analysis revealed the restoration of pancreatic β-cells by the extracts. The LC-MS analysis identified phytocompounds in the extracts belonging to classes of flavonoids, phenolic acids, and fatty acids that are associated with antidiabetic effects. Interestingly, the ethanol extract had a better antidiabetic effect compared to the aqueous extract. In conclusion, the aqueous and ethanol extracts of C. maxima seed possess phytocompounds that can be used to develop novel antidiabetic agents with less severe effects.
INTRODUCTION
Diabetes is a long-term metabolic disease marked by persistently high blood glucose levels due to insufficient insulin secretion and resistance to insulin action, or both [1]. Diabetes is the fastest growing public health concern globally [2]. It is highly linked to microvascular complications such as neuropathy, nephropathy, and retinopathy, as well as macrovascular complications (such as stroke, cerebrovascular disease, coronary artery disease, and steatohepatitis), and sometimes fatalities [3-5]. According to the International Diabetes Federation, 10.5% (536.6 million) of the adult population in the world suffered from diabetes in 2021 [1, 6]. Most of these individuals lived in low- and middle-income countries. It was also estimated that the number will rise to 643 million and 783 million in 2030 and 2045, respectively [1, 6]. Globally, about 6.7 million fatalities in 2021 were directly associated with diabetes [6, 7].
Type I and type II diabetes mellitus are the most common types of diabetes [1]. Type I diabetes is a T-cell-mediated autoimmune disease characterized by destruction of insulin-producing β cells of Langerhans and inadequate insulin production [8]. Type II diabetes results from resistance to insulin action and inadequate insulin secretion by pancreatic β cells [9]. Type II diabetes accounts for approximately 90% of all instances of diabetes, while type I diabetes accounts for about 5 to 10% [6, 10]. Other types of diabetes include monogenic diabetes, gestational diabetes, and secondary diabetes [1]. Diabetes had a significant financial burden amounting to 966 billion USD in 2021 globally [6].
Insulin is primarily responsible for maintaining blood glucose homeostasis. The pancreatic β-cells of the islets of Langerhans are responsible for the synthesis of insulin in the body. After a meal, when blood glucose levels increase, β-cells release insulin into the bloodstream, where it is absorbed by skeletal muscle, adipocytes, and hepatocytes. Insulin reduces or returns blood glucose to normal levels by increasing the absorption of glucose by the adipose tissue and skeletal muscles, as well as inhibiting the liver's endogenous glucose synthesis [11]. In diabetic individuals, the blood sugar levels remain high due to insulin resistance and ineffective action, or both [12].
The management of hyperglycemia in diabetic patients is achieved through lifestyle adjustments and use of synthetic antidiabetic drugs. The lifestyle adjustments include healthy eating and drinking as well as physical exercising [13]. Synthetic antidiabetic drugs are also often prescribed to manage hyperglycemia in individuals. These drugs include injectable medications such as insulin as well as synthetic antidiabetic drugs such as metformin, glibenclamide, and thiazolidinedione, among others [10, 14, 15]. Nevertheless, these medications are associated with severe side effects, including congestive heart failure, gallbladder cancer, gastrointestinal tract toxicity, and hepatotoxicity [9-11]. These data necessitate the need for alternative antidiabetic agents.
Medicinal plants offer great promise for treating and managing several diseases due to the presence of phytochemicals that have therapeutic value. The use of herbal medications is growing popularity as alternative therapeutic agents since they have better therapeutic effects with minimal side effects [19, 20]. Some extracts from medicinal plants have revealed the ability to promote regeneration of the pancreas and enhance insulin secretion [21]. The secondary plant metabolites associated with antidiabetic effects include coumarins, flavonoids, alkaloids, carotenoids, phenolic acids, phytosterols, saponins, tannins, and terpenoids [22]. The seeds of Cucurbita maxima are used by Kenyan communities to manage diabetes. Nevertheless, there was a paucity of empirical evidence to validate this claim. This investigation therefore aimed to determine the phytochemical composition and antidiabetic effects of aqueous and ethanol extracts of C. maxima seed.
MATERIALS AND METHODS
Medicinal sample collection
Fresh parts and fruits of C. maxima (pumpkin) were obtained from a commercial pumpkin farm in Embu, Kenya. The seeds and fresh pumpkin samples were taxonomically identified and authenticated by a taxonomist in the Department of Botany at Jomo Kenyatta University of Agriculture and Technology. The specimen voucher was deposited at the department herbarium (Voucher number 4321). The fruits of the pumpkins were cut and opened to yield approximately 2 kg of the seeds. The seeds were washed with distilled water, air-dried, ground into a fine powder, and packed awaiting extraction.
Aqueous extraction of C. maxima seed
First, 500 g of the powder was put in 2 L of distilled water, and the mixture was left to stand for 2 h with occasional shaking. The mixture was then incubated for one day at 40 °C. The obtained mixture was decanted, centrifuged at 9000 revolutions per minute (rpm) for 10 minutes, and then filtered via Whatman® GF/C glass microfiber filter paper. The filtrate was lyophilized using a freeze dryer (NANBEI freeze dryer: NBJ-10-1, Zhengzhou, China) and the resulting seed extract was pulverized and stored at 4 °C [23].
Ethanol extraction of C. maxima seed
First, 500 g of the seed powder was macerated with 2 L of analytical grade ethanol (Sigma Aldrich, Merck Group, Missouri, United States), shaken for 4 h, and then left to stand for 24 h. The mixture was then filtered using Whatman number 1 filter paper. The extract was concentrated using a vacuum evaporator (Buchi R-200 Rotavapor System, Hampton, United States) at 40 °C. The obtained extract was stored at 4 °C [24].
Animal study
Healthy Wistar rats aged between 8-9 weeks and weighing 160-180 g were utilized in the antidiabetic assay. The rats were acquired and housed at the Institute of Primate Research (IPR), Nairobi. The rats were kept at standard laboratory conditions, fed rodent pellet diet (Unga Group Limited, Kenya), and provided water ad libitum. One week before the experiment, the rats were acclimatized. The experiment was conducted based on the animal ethics and guidelines of the Institute of Primate. Ethical approval of the study was sought from the Institute of Primate Research Ethical Review Committee (ISERC/03/2017) before the commencement of the study.
To induce diabetes, alloxan monohydrate (Sigma Aldrich, Merck Group, St. Louis, Missouri, United States) at 180 mg/kg body weight (bw) was injected (single dose) intraperitoneally in overnight fasted rats. The fasting blood glucose was measured after 72 h. The rats with fasting blood glucose exceeding 11.1 mmol/L were regarded as diabetic and employed in this study.
Thirty-five rats were assigned to 7 groups (5 rats/group): a) Non-diabetic rats (control; received vehicle), b) diabetic rats (alloxan monohydrate at 180 mg/kg), c) glibenclamide (reference drug) at a dosage of 5 mg/kg bw treated to diabetic rats, d-e) aqueous extract at doses of d) 200 and e) 400 mg/kg bw were treated to diabetic rats; and f-g) ethanol extract at doses of f) 200 and g) 400 mg/kg bw were treated to diabetic rats. The treatments were administered orally for 28 days [15].
Fasting blood glucose analysis
The fasting blood glucose in each rat was monitored on 0 h, 2 h, 4 h, 6 h, 24 h, 7 day, 14 day, 21 day, and 28 day. Prior to measuring fasting blood glucose levels, the experimental rats were fasted overnight. The blood sample was obtained by first sterilizing the rat tail using 70% surgical spirit, followed by snipping the tip of the tail. A drop of blood was obtained from the tail of each rat and then a OneTouch Select Plus Simple® Glucose Meter (LifeScan IP Holdings, Malvern, Pennsylvania, United States) was utilized to measure the levels of fasting blood glucose.
Histopathological analysis
The experimental rats were euthanized using diethyl ether on day 29, after which they were dissected, and pancreas was detached. Each pancreas was rinsed with normal saline to remove blood and then transferred immediately to 10% formalin. Subsequently, various alcohol-to-water ratios were used to dehydrate sections of pancreas. The tissues were stored in the following progressive alcohol grades for gradual dehydration: 30% and 50% for 5 to 10 h, 70% for 10 to 12 h, 90% for around thirty minutes, and twenty minutes in 100% alcohol. To facilitate sectioning, the dehydrated tissues were embedded in paraffin wax. After adjusting the section's thickness to 6 micrometers (μm), it was stained with hematoxylin and eosin dye and placed in a neutral deparaffinated xylene (DPX) solution to facilitate microscopic studies at magnification of x400.
Liquid chromatography-mass spectrometry analysis
One gram of each extract was weighed, dissolved in 1 mL in double distilled water/acetonitrile, 95/5 (v/v) with 0.1 % formic acid (Sigma Aldrich, Merck Group, St. Louis, Missouri, United States), centrifuged at 15,000 rpm for 2 minutes, and then transferred into clean labeled vials. From each extract, a stock solution of 1 mg/1 mL was prepared and 20 µl of the extract was injected into a liquid chromatography-mass spectrometry (LC-MS)-electrospray ionization (ESI) vial. The operating conditions were as follows: a quaternary LC pump (Model 1200, Agilent Technology, Palo Alto, California, United States) coupled to Agilent MSD 6120-Triple quadruple MS with an electrospray source (Agilent Technology, Palo Alto, California, United States) was utilized. ChemStation software (Hewlett-Packard) was used to control the system. Reversed-phase liquid chromatography was conducted on an Agilent Technology 1200 infinite series, Zorbax SB C 18 column, 2.1 x 50 mm, 1.8 µm (Phenomenex, Torrance, California, United States) using the following gradient program: 0 minute, 5 % B; 0-5 minutes, 5-50 % B; 5-10 minutes, 50-80 % B; 10-15 minutes, 80-100 % B; 15-25 minutes, 100 % B; 25-30 minutes, 5 % B; 30-35 minutes, 5 % B (Water; Acetonitrile). The flow rate was maintained at 1 mL/min.
A scan range of 100 to 1500 m/z was used to collect data in both positive and negative ion modes. Every ion had a dwell period of 50 milliseconds. The mass spectrometer had the following additional settings: capillary voltage, 3.0 kV; cone voltage, 70 V; extract voltage, 5 V; RF voltage, 0.5 V; source temperature, 110 ºC; nitrogen gas temperature for desolvation, 380 °C; and 400 L/nitrogen gas flow. Serial dilutions (1-100 ng/µL) of rutin hydrate standard were analyzed using LC-MS-ESI in full scan mode to generate the following linear calibration curve: [y = 5578.4x − 39094 (R2 = 0.9990)]. The equation served as a basis for external quantification. Automated quantification of the compounds was acquired in reference to the calibration curve and instrumentally tabulated against the exact mass ions.
Statistical analysis
Raw data was analyzed using GraphPad Prism version 9. The mean ± standard error of the mean (SEM) was used to express descriptive statistics. An inferential statistic, one-way ANOVA (analysis of variance), was employed to test for statistical differences among different treatment groups. In case of statistical differences, the data was subjected to Tukey’s multiple comparisons. To compare the effects of the two extracts, an independent t-test was computed. The level of significance was set at p less than 0.05.
RESULTS
Effects of aqueous and ethanol seed extracts on levels of fasting blood glucose in rats
The aqueous seed extract of C. maxima at 200 and 400 mg/kg bw demonstrated an antidiabetic effect following alloxan-induced diabetes in rats (Table 1). The diabetic control rats had higher levels of fasting blood glucose from 2 h of the experiment. Treatment with aqueous seed extract at 200 and 400 mg/kg bw substantially reduced the fasting blood glucose levels relative to the levels (p<0.05) in diabetic control rats. The levels of fasting blood glucose in normal control rats remained remarkably low throughout the experiment (p<0.05; Table 1). The baseline of all studied groups did not vary significantly (p>0.05) before the induction of diabetes (Table 1).
The antidiabetic effect of aqueous seed extract at the two doses did not differ significantly (p>0.05) in 2 h. Nevertheless, in 4, 6, and 24 h, the extract dose of 400 mg/kg bw significantly lowered the blood glucose levels compared to the levels noted (p<0.05) at 200 mg/kg bw (Table 1). In 2, 4, and 6 h, the fasting blood glucose levels in glibenclamide-treated rats were substantially lower relative to levels noted (p<0.05) in extract-treated rats at the two studied doses, although in 24 h, the blood glucose levels were insignificant compared to levels of the higher dose of the extract (p>0.05). The blood glucose levels in diabetic control rats were significantly higher relative to levels observed in other treatment groups in 4, 6, and 12 h (p<0.05). The non-diabetic control rats had considerably lower fasting blood glucose levels than those recorded in other treatment groups in 0, 2, 4, 6, and 24 h (p<0.05; Table 1).
On days 7 and 21, the levels of fasting blood glucose in aqueous seed extract of C. maxima at 400 mg/kg bw were substantially lower than those at 200 mg/kg bw (p<0.05), although the levels at the two doses were insignificant (p>0.05) on days 14 and 28 (Table 1). The levels of fasting blood glucose in glibenclamide-treated rats were significantly lower on days 7, 21, and 28 (p<0.05). On days 7, 14, 21, and 28, the fasting blood glucose levels in diabetic control rats were considerably greater compared to those seen in the other treatment groups (p<0.05). Nevertheless, in the same period, the fasting blood glucose levels were substantially lower in non-diabetic control rats (p<0.05; Table 1).
The rats that were administered with ethanol seed extract of C. maxima at 200 and 400 mg/kg bw noted a reduction in the levels of fasting blood glucose on alloxan-induced diabetes in rats (Table 2). The levels of fasting blood glucose at the baseline did not differ considerably in all the studied groups (p>0.05). From 2 h of the experiment, the diabetes control rats had considerably higher levels of fasting blood glucose (p<0.05). The non-diabetic rats noted unchanged fasting blood glucose levels in the entire experiment (p>0.05; Table 1).
In 2 and 4 h of the study, the fasting blood glucose levels of two doses of ethanol seed extract were nonsignificant in diabetic rats (p>0.05). Nonetheless, in 6 and 24 h, the levels of blood glucose in rats that were administered ethanol seed extract at 400 mg/kg bw were significantly lower than those noted (p<0.05) at 200 mg/kg bw (Table 2). The levels of fasting blood glucose in glibenclamide-treated rats statistically matched those of rats that received ethanol seed extract at the two doses in 2 and 4 h (p>0.05). Similarly, in 6 and 24 h, the levels of fasting blood glucose in animals treated with glibenclamide were statistically similar to the levels noted in animals treated with ethanol seed extract (p>0.05) at 400 mg/kg bw. The levels of fasting blood glucose in diabetes control rats remained substantially higher compared to other treatment groups in 2, 4, 6, and 24 h (p<0.05). Contrarily, the non-diabetic control rats had substantially lower levels of fasting blood glucose relative to the levels (p<0.05) of the other treatment groups in all the studied times (Table 2).
On days 14, 21, and 28, the levels of blood glucose in animals treated with ethanol seed extract were statistically insignificant (p>0.05) at the two studied doses (Table 2). On days 7 and 14, the effect of glibenclamide on the fasting blood glucose levels did not differ significantly relative to the levels seen in extract-treated rats at the two doses (p>0.05). The fasting blood glucose levels were considerably greater in diabetes control rats compared to levels noted in (p<0.05) other treatment groups on days 7, 14, 21, and 28. In the same period, the non-diabetic control rats revealed substantially lower fasting blood glucose levels relative to levels observed in the other studied groups (p<0.05; Table 2).
Table 1. Effect of C. maxima aqueous seed extract on levels of fasting blood glucose on alloxan-induced diabetes in rats.
Table 2. Effect of C. maxima ethanol seed extracts on levels of fasting blood glucose on alloxan-induced diabetes in rats.
In comparison, the effects of aqueous and ethanol seed extracts of C. maxima at 200 mg/kg bw on fasting blood glucose levels did not differ significantly in 0 h (p>0.05). Nevertheless, at the same dose, the levels of fasting blood glucose were considerably lower in rats that received ethanol extract relative to the levels noted in rats that received aqueous extract in 2, 4, 6, and 24 h (p<0.05; Figure 1). At 400 mg/kg bw, the effect of the two extracts did not differ significantly on fasting blood glucose levels in 0 and 2 h (p>0.05). Nonetheless, the fasting blood glucose levels in rats that were administered ethanol seed extract at 400 mg/kg bw were substantially lower relative to levels observed in rats that received aqueous seed extract in 4, 6, and 24 h (p<0.05; Figure 1).
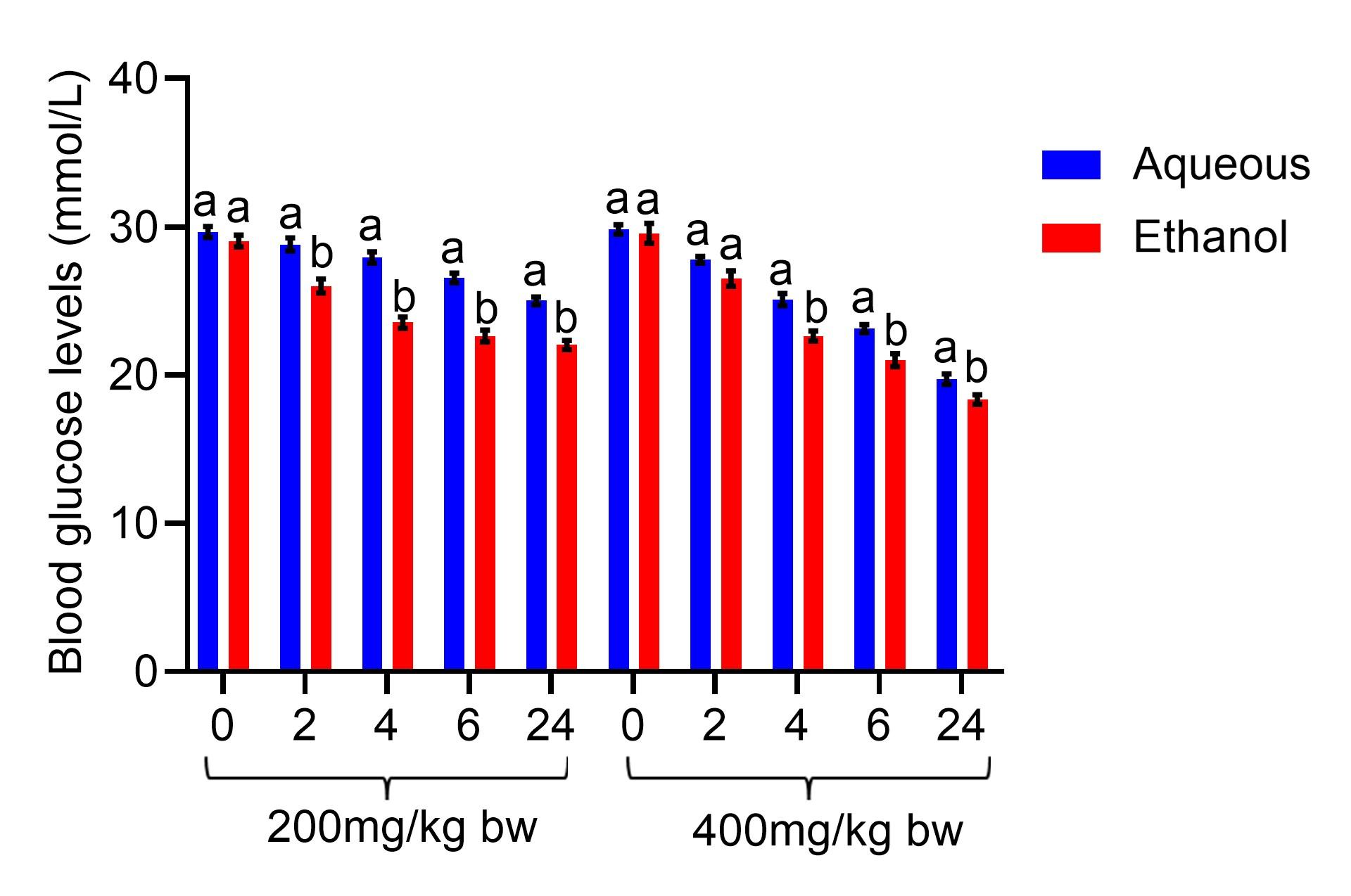
The fasting blood glucose levels were also compared on the treatment days. The diabetic rats that were administered ethanol seed extract at 200 mg/kg bw revealed a substantial decline in their fasting blood glucose levels compared to the levels seen in rats that received aqueous seed extract on days 7, 14, 21, and 28 of the experiment (p<0.05; Figure 2). At 400 mg/kg bw, treatment with the ethanol seed extract of C. maxima substantially lowered levels of fasting blood glucose levels compared to the levels noted in diabetic rats that received aqueous seed extract on days 7, 14, 21, and 28 of the experiment (p<0.05; Figure 2).
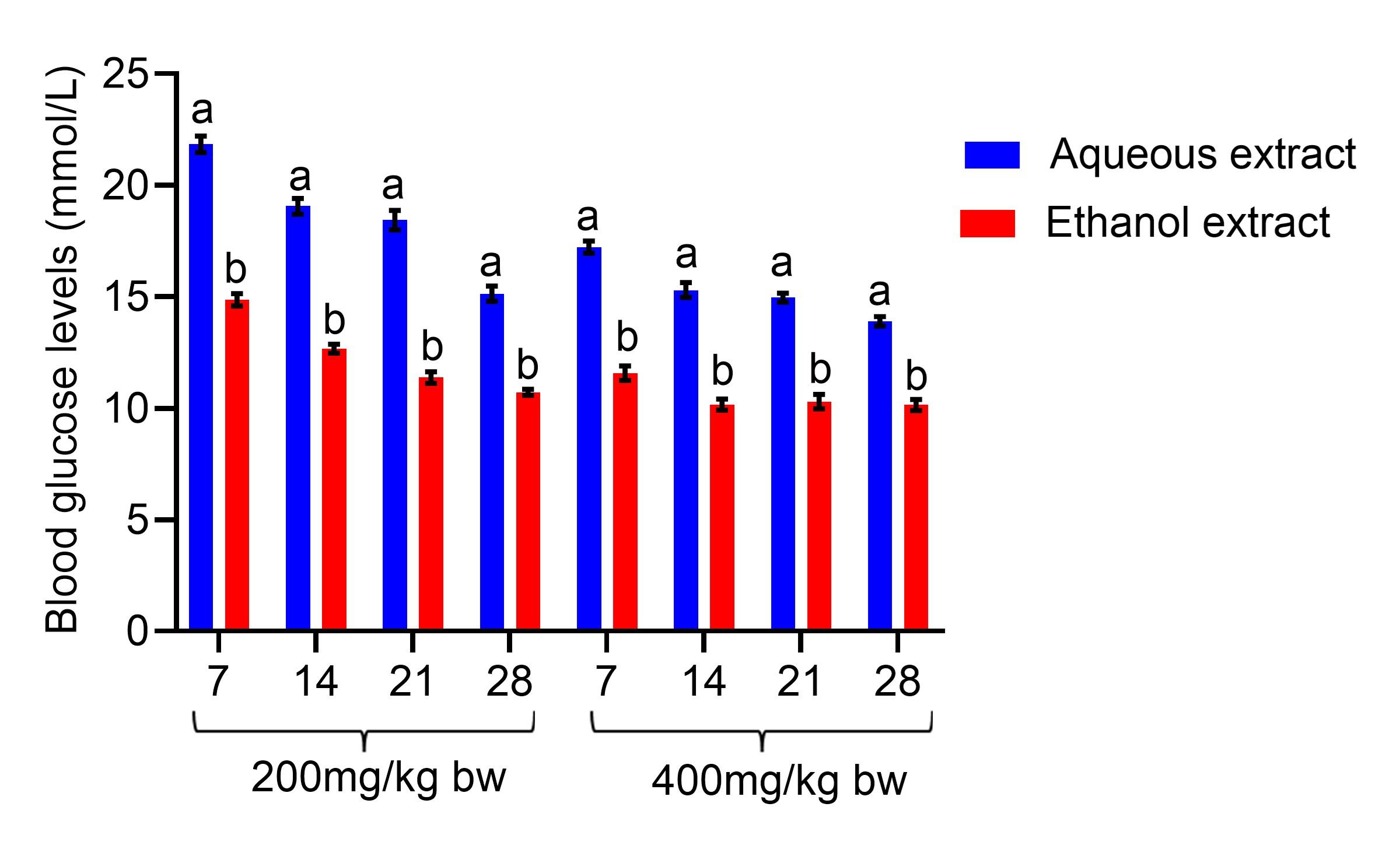
Effect of the extracts on histopathology of the pancreas in diabetic rats
The histopathology of the pancreas in normal control rats had normal β-cells of islets of Langerhans (Figure 3). The induction of diabetes in rats resulted in degeneration of pancreatic β-cells, pancreatic inter-lobular fibrosis, and massive infiltration of neutrophils enclosed within an eosinophilic fibrous capsule. However, treatment with the reference drug, glibenclamide and the two extracts revealed restoration of the pancreatic β-cells. At 200 mg/kg bw of the two extracts, the histopathology of the pancreas showed partly mild regeneration of pancreatic β-cells. At 400 mg/kg bw, the effects of the two extracts revealed a moderate regeneration of the β-cells (Figure 3).
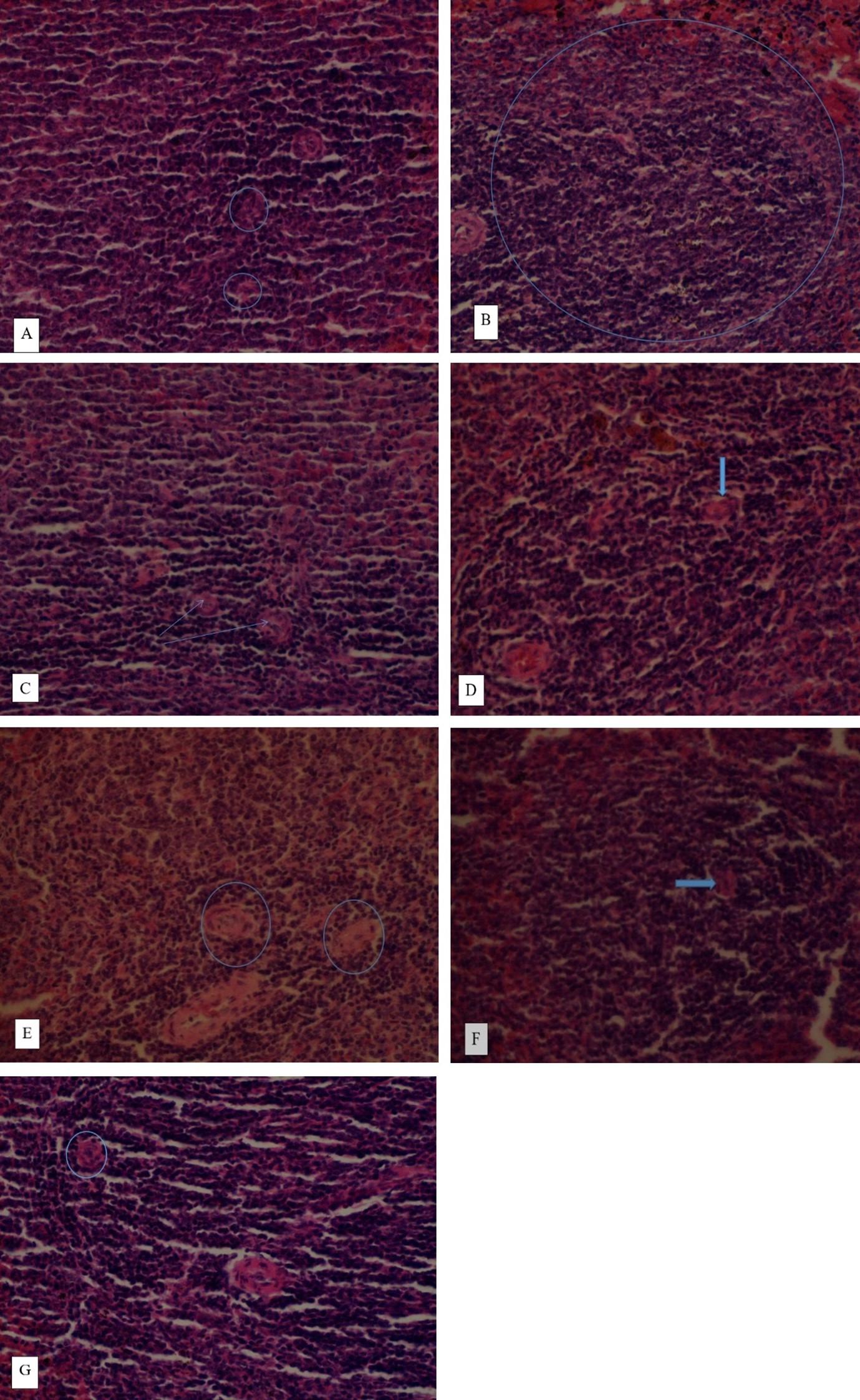
Phytochemical composition of seed extracts of C. maxima
The LC-MS analysis of the two extracts identified phytocompounds that belonged to classes of phenolic acids, flavonoids, and fatty acids (Figures 4 and 5). The aqueous extract detected phenolic acids such as 4-hydroxybenzoic acid (retention time in minutes (RT) = 15.77), concentration in µg/mg (Conc.) = 1.67), protocatechuic acid (RT of 15.36, Conc. = 0.29), 4-hydroxycinnamic acid (RT of 2.98, Conc. = 68.67 ), vanillic acid (RT of 14.89, Conc. = 0.03), gallic acid (RT = 2.96, Conc. = 68.67), quinic acid (RT =10.52, Conc. = 0.08), ferulic acid (RT = 14.82), rosmarinic acid (RT = 13.8), 3-feruloylquinic acid (RT = 13.63) and chicoric acid (RT = 13.7; Conc. = 0.03) (Figure 4).
Flavonoids such as biochanin B (RT = 10.52, Conc. = 0.60), calconaringenin (RT = 14.8, Conc. = 0.64), eriodictyol (RT = 14.9, Conc. = 0.64), taxifolin (RT = 14.33, Conc. = 0.76), epigallocatechin (RT = 12.82, Conc. = 0.02), cirsiliol (RT = 14.62, Conc. = 0.21), genistin (RT = 12.01, Conc. = 0.54), Kaempferol-3-glucorhamnoside (RT = 13.8; Conc. = 0.03), diosimin (RT = 12.45, Conc. = 0.02) and myricetin (RT = 10.89, Conc. = 0.33, negative mode) were detected in the aqueous extract (Figure 4). Fatty acids, including palmitoleic acid (RT = 10.42, Conc. = 0.42), linoleic acid (RT = 14.3, Conc. = 0.17, negative mode) and oleic acid (RT = 11.64, Conc. = 2.41, negative mode) were also detected in LC-MS analysis of the aqueous extract (Figure 4).
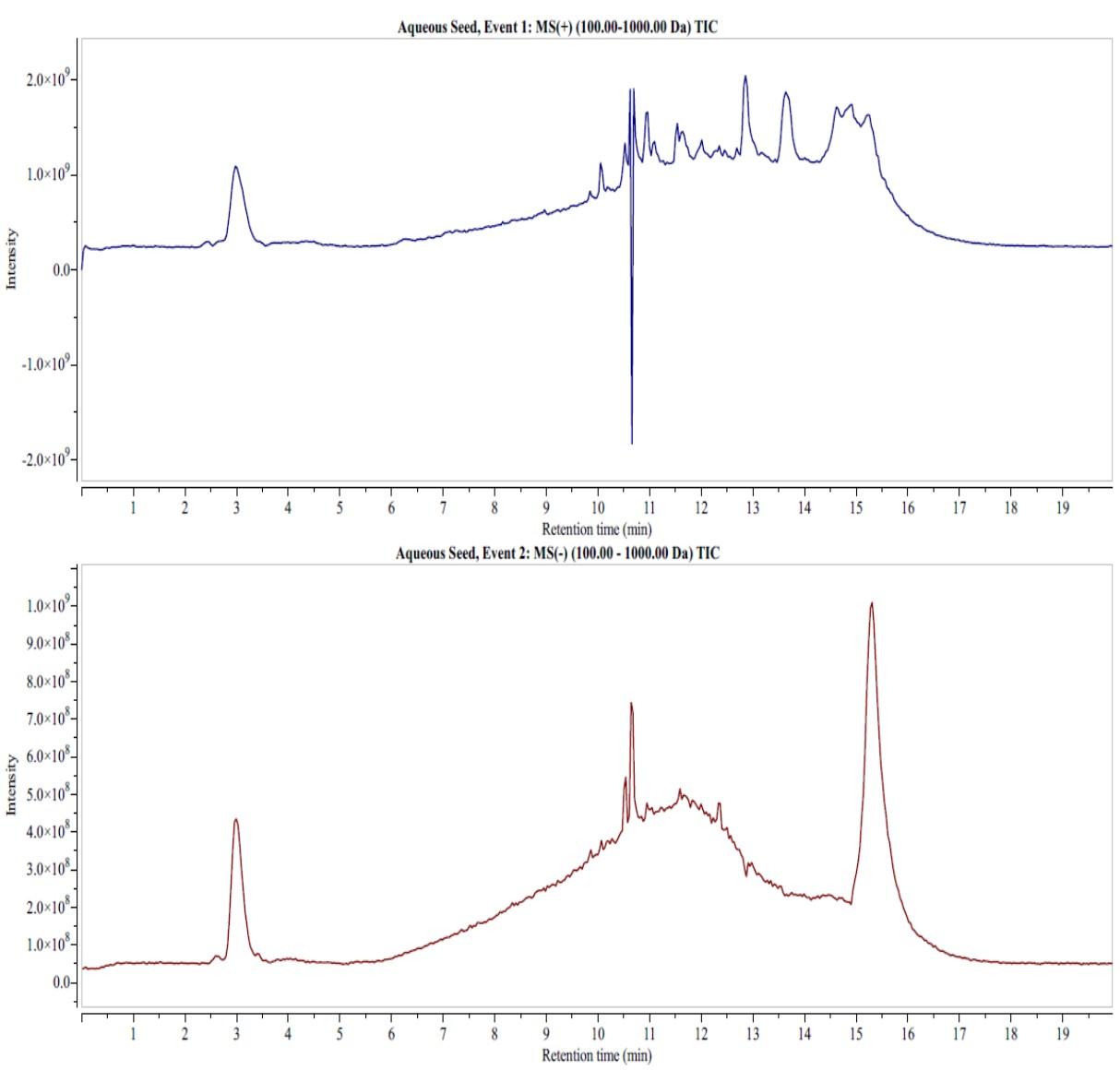
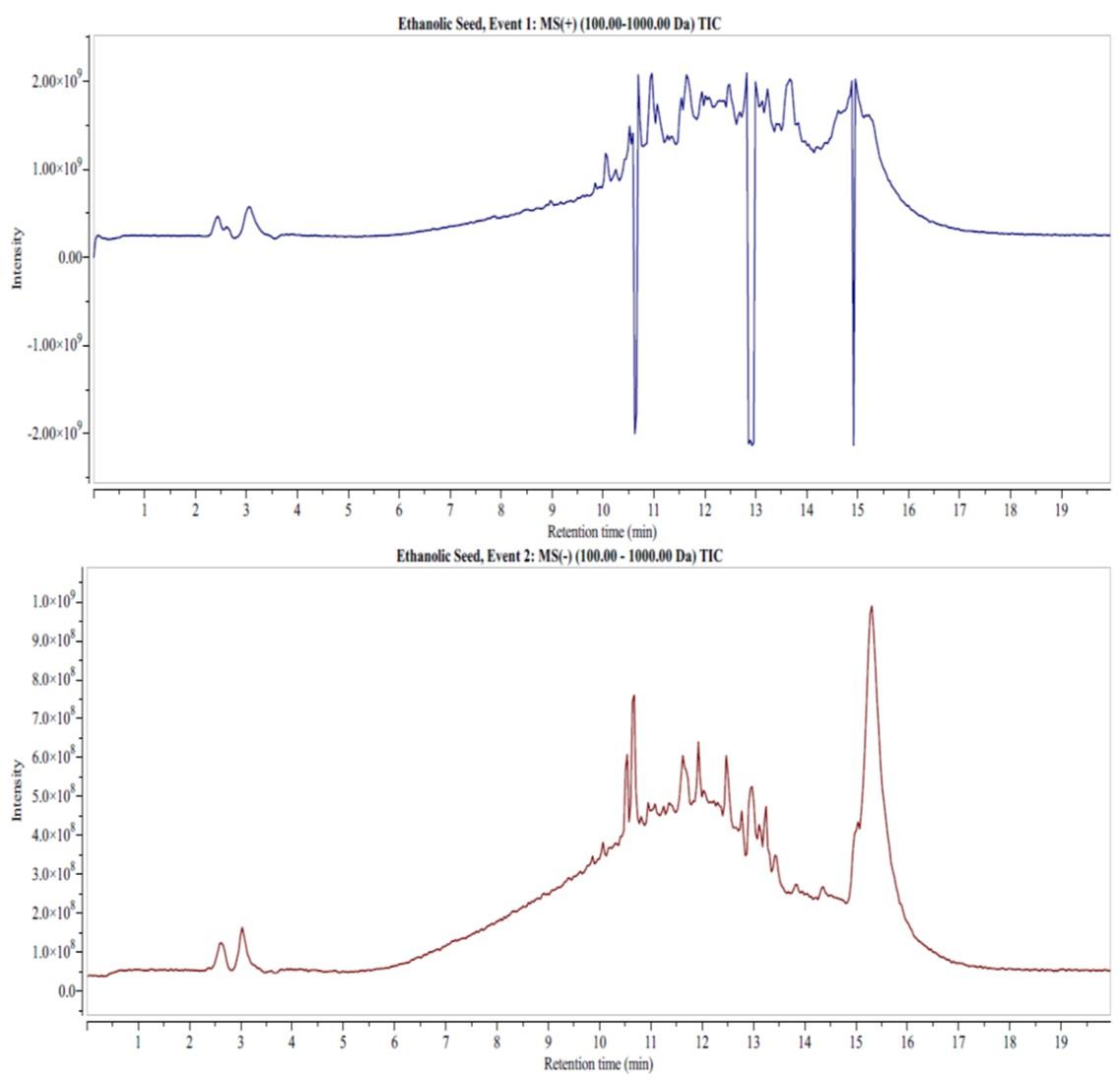
DISCUSSION
In this study, the effects of aqueous and ethanol seed extracts at doses of 200 and 400 mg/kg bw of C. maxima attenuated elevated fasting blood glucose levels, including restoration of damaged pancreatic β-cells following alloxan-induced diabetes in rats. The LC-MS analysis of the two extracts detected phytocompounds that belonged to the classes of phenolic acids, flavonoids, and fatty acids. These plant secondary metabolites have been documented to possess antidiabetic effects [26].
After diabetic rats were administered the two extracts for 28 days, the blood glucose levels gradually lowered throughout the treatment period, suggesting that a higher dosage had a more potent antidiabetic effect. Various research studies have also reported the antidiabetic effects of medicinal plant extracts following alloxan-induced diabetes in animal models. A study by Sharma et al. [27] found that C. maxima ethanol seed extract had an antidiabetic effect on streptozotocin-induced diabetes in rats. Kushawaha et al. [28] revealed that C. maxima aqueous seed extract had an antidiabetic effect on streptozotocin-induced diabetes in rats. Atta et al. [29] demonstrated that C. maxima fruit methanol extract attenuated streptozotocin-induced diabetes in rats. Suwannapong et al. [30] reported that pulp extract of C. maxima had an antidiabetic effect on streptozotocin-induced diabetes in rats.
Alloxan, an unstable organic compound, is commonly utilized as a diabetogenic agent in experimental research [25]. Alloxan is widely recognized for its ability to damage pancreatic β-cells of the islets of Langerhans, which consequently impairs insulin secretion, thereby causing hyperglycemia [31]. Alloxan-induced diabetes is caused by two different mechanisms: first, it selectively inhibits glucose-induced insulin secretion by specifically inhibiting glucokinase, the β-cell's glucose sensor; second, it produces reactive oxygen species (ROS) that destroy insulin-producing pancreatic β-cells in the pancreas, leading to reduced synthesis and release of insulin, thereby causing hyperglycemia [32].
Glibenclamide is highly prescribed to manage diabetes mellitus [33], hence the choice of this study. Glibenclamide is classified as part of the second-generation of sulfonylurea. Its mechanism of action involves inhibiting adenosine triphosphate-sensitive K+ channels of pancreatic β-cells, which increases insulin secretion and sensitivity of insulin-dependent organs, such as the muscles, adipose tissues, and liver, leading to the utilization of glucose [34].
Antidiabetic effects of medicinal plants have been linked to a number of mechanisms, including stimulation of insulin secretion by the pancreas, suppression of enzymes that break down carbohydrates, increased expression of glycogen-regulatory enzymes in the liver, and enhanced uptake of glucose by skeletal muscles and adipocytes [35]. The effect of two extracts in this study could have occurred through a variety of mechanisms, including slowing down the gut's absorption of carbohydrates, boosting the production of insulin by potentially regenerated β-cells, reducing the endogenous synthesis of glucose by the liver, or stimulating the absorption of glucose by skeletal muscles and adipocytes [19, 22]. Also, the restoration in the integrity of the pancreas tissue as shown in this study could have possibly enhanced the function of regenerated pancreatic β-cells, thus increasing the secretion of insulin [31].
In this study, phytochemicals associated with antidiabetic effects, such as phenolic acids, flavonoids, and fatty acids, were detected using LC-MS analysis. The flavonoids included biochanin B, calconaringenin, eriodictyol, taxifolin, epigallocatechin, cirsiliol, genistin, kaempferol-3-glucorhamnoside, diosimin, myricetin, leutolin, and apigenin. Biochanin B exerts its antidiabetic effect through stimulation of β-cells to secrete insulin, as well as reducing the activity of gluconeogenesis enzymes and enhancing glycolytic enzymes [36]. Chalconaringenin (isosalipurpol or naringenin chalcone) inhibits the absorption of glucose in the intestines as well as renal glucose reabsorption, thus causing an antidiabetic effect [37]. Eriodictyol has demonstrated its antihyperglycemic effect through inhibition of lipid peroxidation caused by ROS [38].
Taxifolin (dihydroquercetin) exerts its antidiabetic effect by promoting the translocation of glucose transporter 4 (GLUT4), which is involved in insulin-regulated glucose absorption in fat and muscle cells [39]. Genistein has shown its antidiabetic effect through enhanced β-cell proliferation, suppression of production of hepatic glucose, and reduction in β-cell apoptosis [40]. Myricetin inhibits α-glucosidase and α-amylase, prevents digestion of carbohydrates, and enhances expression of GLUT4 to exert its antidiabetic effect [41]. Luteolin has been shown to reverse hyperlipidemia and oxidative stress, thus exerting its hyperglycemia effect [29, 30]. Apigenin enhances the release of insulin from the pancreas and stimulates the metabolism of glucose and its delivery to peripheral tissues [44].
The phenolic acids that were detected using LC-MS included 4-hydroxybenzoic acid, vanillic acid, gallic acid, quinic acid, protocatechuic acid, 4-hydroxycinnamic acid, ferulic acid, rosmarinic acid, 3-feruloylquinic acid, chicoric acid, and syringic acid. 4-Hydroxybenzoic acid has been shown to exert its antidiabetic effect by enhancing peripheral glucose consumption [45]. Gallic acid exerts its antidiabetic effect by increasing glucose consumption, including the reduction in the synthesis of reactive oxygen species and enhanced glucose uptake [33, 34]. 4-Hydroxybenzoic acid also exerts its antihyperglycemic effect through enhanced insulin secretion and expression of GLUT4 [48]. Chicoric acid increases insulin secretion and enhances glucose uptake in muscle cells to exert its antidiabetic effect [49]. 4-Hydroxycinnamic acid stimulates insulin secretion in the pancreas, inhibits hepatic gluconeogenesis, enhances glucose uptake, improves pancreatic β-cell functionality, and delays the digestion of carbohydrates and absorption of glucose [37, 38].
Ferulic acid restores pancreatic architecture and modulates important diabetogenic actions to promote glucose homeostasis [52]. Rosmarinic acid has been documented to possess an antidiabetic effect through regeneration of pancreatic β-cells, increased glycogen content in skeletal muscle, enhanced activity of glycogen synthase, and suppression of glycogen phosphorylase [40, 41]. Syringic acid exhibits its antidiabetic effect by lowering the activities of glucose-6 phosphatase and fructose-1,6-bisphosphatase, as well as enhancing the activities of glycolytic enzymes to modulate carbohydrate metabolism [55].
Fatty acids that were identified using LC-MS analysis included palmitoleic acid, linoleic acid, and oleic acid. Palmitoleic acid (omega-7 monounsaturated fatty acid) exerts its antidiabetic effect by enhancing insulin sensitivity [56]. Linoleic acid (omega-6 fatty acid) exerts its antidiabetic effect through inhibition of the protein tyrosine phosphatase linked to insulin resistance [57]. Oleic acid (omega-9 fatty acid) protects against insulin resistance through the regulation of genes associated with the phosphatidylinositol 3-kinases signaling pathway [58].
The ethanol extract had a better antidiabetic effect than the aqueous extract, although it had fewer phytochemicals. This could be due to the ability of ethanol to extract both polar and non-polar compounds. The non-polar compounds could also have increased the antidiabetic effect.
CONCLUSIONS
This investigation concluded that ethanol and aqueous seed extracts of C. maxima at dosages of 200 and 400 mg/kg bw have potent antidiabetic effects following alloxan-induced diabetes in rats. The antidiabetic effect of the two extracts was associated with the phytocompounds that were detected using LC-MS analysis. The findings of this study can be utilized as a lead in developing novel antidiabetic drugs.
ACKNOWLEDGMENTS
The authors would like to acknowledge the Government of Kenya through the National Research Fund for their financial support (Grant No. NRF/PhD/01/24). We would also like to sincerely thank the Department of Animal Sciences, Institute of Primate Research, for the provision, handling, and care of the laboratory animals. We are also grateful to Ms. Esther Kagasi, Mr. Halkano Molu, Ms. Pauline Otoli, Mr. Thomas Adino, and Mr. Sammy Poghon from the Institute of Primate Research, Kenya, for their technical support.
AUTHOR CONTRIBUTIONS
PN, RW, and HO conceived and designed the study. SM and PN conducted experiments and analyzed data. RW, HO, SM, AM, and LA interpreted the data and supervised the project. All authors participated in drafting the manuscript.
CONFLICTS OF INTEREST
There is no conflict of interest among the authors.
References
- [1]Kumar A, Gangwar R, et al. Prevalence of diabetes in India: A review of IDF diabetes atlas 10th edition. Current diabetes reviews. 2024;20(1):105-14.
- [2]Hossain MJ, Al‐Mamun M, et al. Diabetes mellitus, the fastest growing global public health concern: Early detection should be focused. Health Science Reports. 20247(3):e2004.
- [3]Arokiasamy P, Salvi S, et al. Global burden of diabetes mellitus. InHandbook of global health 2021 (pp. 1-44). Cham: Springer International Publishing.
- [4]Kropp M, Golubnitschaja O, et al. Diabetic retinopathy as the leading cause of blindness and early predictor of cascading complications—risks and mitigation. Epma Journal. 2023;14(1):21-42.
- [5]WHO. Diabetes 2023. https://www.who.int/news-room/fact-sheets/detail/diabetes. Retrieved 5th June 2024.
- [6]International Diabetes Federation (IDF). Diabetes around the world in 2021. https://idf.org/about-diabetes/diabetes-facts-figures. Retrieved 2nd July 2024.
- [7]Sun H, Saeedi P, et al. IDF Diabetes Atlas: Global, regional and country-level diabetes prevalence estimates for 2021 and projections for 2045. Diabetes Research and Clinical Practice. 2022;183:109119.
- [8]Bawatneh A, Darwish A, et al. Identification of gene mutations associated with type 1 diabetes by next-generation sequencing in affected Palestinian families. Frontiers in Genetics. 2024;14:1292073.
- [9]Padhi S, Nayak AK, et al. Type II diabetes mellitus: a review on recent drug-based therapeutics. Biomedicine & Pharmacotherapy. 2020;131:110708.
- [10]Ojo OA, Ibrahim HS, et al. Diabetes mellitus: From molecular mechanism to pathophysiology and pharmacology. Medicine in Novel Technology and Devices. 2023;19:100247.
- [11]Galicia-Garcia U, Benito-Vicente A, et al. Pathophysiology of type 2 diabetes mellitus. International Journal of Molecular Sciences. 2020;21(17):6275.
- [12]Lee SH, Park SY, Choi CS. Insulin resistance: from mechanisms to therapeutic strategies. Diabetes & Metabolism Journal. 2022;46(1):15.
- [13]American Diabetes Association. 4. Lifestyle management: standards of medical care in diabetes—2018. Diabetes care. 2018;41(1):38-50.
- [14]Goyal R, Singhal M, et al. Type 2 diabetes. StatPearls [Internet]. 2023.
- [15]Gupta A, Yadav A, et al. Diabetes Mellitus: Understanding the Pathophysiology and Therapeutic Strategies. Journal of Clinical Advances and Research Reviews. 2024;1(1), 57-63.
- [16]Corcoran, C, Tibb F. “Metformin.” StatPearls [Internet]. StatPearls Publishing, 2023.
- [17]Costello, Ryan A., Samar Nicolas, and Abhijit Shivkumar. “Sulfonylureas.” StatPearls [Internet]. StatPearls Publishing, 2023.
- [18]Eggleton JS, Jialal I. Thiazolidinediones. StatPearls [Internet]. Treasure Island (FL): StatPearls Publishing. 2023.
- [19]Badsha SA, Islam R, et al. Effect of Gynura procumbens leaf extract with biological nanoparticles on streptozotocin-induced hyperglycemia in a rat model. Journal of Advanced Biotechnology and Experimental Therapeutics. 2024; 7(1):255-265.
- [20]Ayobami AL, Kade EA, et al. Anti-diabetic Potential of Aqueous Extract of Moringa oleifera, Ocimum gratissimum and Vernonia amygdalina in Alloxan-Induced Diabetic Rats. bioRxiv. 2020: 2(3):78-85..
- [21]Jacob B, Narendhirakannan RT. Role of medicinal plants in the management of diabetes mellitus: a review. 3 Biotech. 2019;9:1-7.
- [22]Yedjou CG, Grigsby J, et al. The management of diabetes mellitus using medicinal plants and vitamins. International journal of molecular sciences. 2023;24(10):9085.
- [23]Ojuade FI, Olorundare OE, et al. Antidiabetic and antihyperlipidemic effects of aqueous extract of Parquetina nigrescens in streptozotocin–nicotinamide induced type 2 diabetic rats. Heliyon. 2021;7(6):e07363.
- [24]Kouamé NG, Koffi C, et al. Comparative antidiabetic activity of aqueous, ethanol, and methanol leaf extracts of Persea americana and their effectiveness in type 2 diabetic rats. Evidence‐Based Complementary and Alternative Medicine. 2019;2019(1):5984570.
- [25]Woldekidan S, Mulu A, et al. Evaluation of antihyperglycemic effect of extract of Moringa stenopetala (Baker f.) aqueous leaves on alloxan-induced diabetic rats. Diabetes, Metabolic Syndrome and Obesity. 2021:185-92.
- [26]Sukhikh S, Babich O, et al. Antidiabetic properties of plant secondary metabolites. Metabolites. 2023;13(4):513.
- [27]Sharma A, Sharma AK, et al. Antidiabetic and antihyperlipidemic activity of Cucurbita maxima Duchense (pumpkin) seeds on streptozotocin induced diabetic rats. Journal of Pharmacognosy and Phytochemistry. 2013;1(6):108-16.
- [28]Kushawaha DK, Yadav M, et al. Evidence based study of antidiabetic potential of C. maxima seeds–In vivo. Journal of traditional and complementary medicine. 2017 Oct 1;7(4):466-70.
- [29]Atta AH, Saad SA, et al. Cucumis sativus and Cucurbita maxima extract attenuate diabetes-induced hepatic and pancreatic injury in a rat model. Journal of Physiology & Pharmacology. 2020;71(4).
- [30]Suwannapong A, Talubmook C, et al. Evaluation of Antidiabetic and Antioxidant Activities of Fruit Pulp Extracts of Cucurbita moschata Duchesne and Cucurbita maxima Duchesne. The Scientific World Journal. 2023;2023(1):1124606.
- [31]Ayobami AL, Kade EA, et al. Anti-diabetic Potential of Aqueous Extract of Moringa oleifera, Ocimum gratissimum and Vernonia amygdalina in Alloxan-Induced Diabetic Rats. bioRxiv. 2020:2020-08.
- [32]Ibrahim AA, Abdussalami MS, et al. Antidiabetic effect of aqueous stem bark extract of Parinari macrophylla in alloxan-induced diabetic Wistar rats. Future Journal of Pharmaceutical Sciences. 2021;7(1):164.
- [33]Ahmed N, Abo-zeid Y, et al. Strategies adopted to improve bioavailability of Glibenclamide: Insights on novel delivery systems. Journal of Advanced Pharmacy Research. 2023;7(1):35-49.
- [34]Mendes CP, Postal BG, et al. Synthesis of a novel glibenclamide-pioglitazone hybrid compound and its effects on glucose homeostasis in normal and insulin-resistant rats. Bioorganic Chemistry. 2021;114:105157.
- [35]Ifebi HM, Onyegbule FA, et al. Evaluation of antidiabetic property of Sansevieria liberica Gerald and Labroy (Dracaenaceae) leaf using alloxan induced diabetes model. Nigerian Journal of Pharmaceutical Research. 2021;16:73-84.
- [36]Sarfraz I, Rasul A, et al. Biochanin A and biochanin B. InA Centum of Valuable Plant Bioactives. Academic Press. 2021:563-588.
- [37]Den Hartogh DJ, et al. Antidiabetic properties of naringenin: A citrus fruit polyphenol. Biomolecules. 2019;9(3):99.
- [38]AlTamimi JZ, AlFaris NA, et al. Protective effect of eriodictyol against hyperglycemia-induced diabetic nephropathy in rats entails antioxidant and anti-inflammatory effects mediated by activating Nrf2. Saudi Pharmaceutical Journal. 2023;31(11):101817.
- [39]Kondo S, Adachi SI, et al. Antidiabetic effect of taxifolin in cultured L6 myotubes and type 2 diabetic model KK-ay/Ta mice with hyperglycemia and hyperuricemia. Current Issues in Molecular Biology. 2021;43(3):1293-306.
- [40]Abbasi E, Khodadadi I. Antidiabetic effects of genistein: Mechanism of action. Endocrine, Metabolic & Immune Disorders-Drug Targets. 2023;23(13):1599-610.
- [41]Niisato N, Marunaka Y. Therapeutic potential of multifunctional myricetin for treatment of type 2 diabetes mellitus. Frontiers in Nutrition. 2023;10:1175660.
- [42]Alam O, Al-Keridis LA, et al. Evaluation of antidiabetic effect of luteolin in STZ induced diabetic rats: Molecular docking, molecular dynamics, in vitro and in vivo studies. Journal of Functional Biomaterials. 2023;14(3).
- [43]Sangeetha R. Luteolin in the management of type 2 diabetes mellitus. Current Research in Nutrition and Food Science Journal. 2019;7(2):393-8.
- [44]Barky A, Ezz A, et al. The Potential role of apigenin in diabetes mellitus. International Journal of Clinical Case Reports and Reviews. 2020;3(1):32.
- [45]Lee H, Lee J. Anti‐diabetic effect of hydroxybenzoic acid derivatives in free fatty acid‐induced HepG2 cells via miR‐1271/IRS1/PI3K/AKT/FOXO1 pathway. Journal of Food Biochemistry. 2021;45(12):e13993.
- [46]Xu Y, Tang G, et al. Gallic acid and diabetes mellitus: its association with oxidative stress. Molecules. 2021;26(23):7115.
- [47]Uddin SJ, Afroz M, et al. A systematic review on anti-diabetic and cardioprotective potential of gallic acid: a widespread dietary phytoconstituent. Food Reviews International. 2022;38(4):420-39.
- [48]Rosiles-Alanis W, Zamilpa A, et al. 4-Hydroxybenzoic Acid and β-Sitosterol from Cucurbita ficifolia Act as Insulin Secretagogues, Peroxisome Proliferator-Activated Receptor-Gamma Agonists, and Liver Glycogen Storage Promoters: In Vivo, In Vitro, and In Silico Studies. Journal of Medicinal Food. 2022;25(6):588-596.
- [49]Alam S, Sarker MM, et al. Antidiabetic phytochemicals from medicinal plants: prospective candidates for new drug discovery and development. Frontiers in Endocrinology. 2022;13:800714.
- [50]Adisakwattana S. Cinnamic acid and its derivatives: mechanisms for prevention and management of diabetes and its complications. Nutrients. 2017;9(2):163.
- [51]Singh B, Kumar A, et al. Protective effect of vanillic acid against diabetes and diabetic nephropathy by attenuating oxidative stress and upregulation of NF‐κB, TNF‐α and COX‐2 proteins in rats. Phytotherapy Research. 2022;36(3):1338-52.
- [52]Salau VF, Erukainure OL, et al. Ferulic acid improves glucose homeostasis by modulation of key diabetogenic activities and restoration of pancreatic architecture in diabetic rats. Fundamental & Clinical Pharmacology. 2023;37(2):324-39.
- [53]Ramalingam S, Karuppiah M, et al. Antihyperglycaemic potential of rosmarinic acid attenuates glycoprotein moiety in high-fat diet and streptozotocin-induced diabetic rats. All Life. 2020;13(1):120-30.
- [54]Silva ML, Lucarini R, et al. Hypoglycemic effect of rosmarinic acid-rich infusion (RosCE) from Origanum vulgare in alloxan-induced diabetic rats. Natural Product Research. 2022;36(17):4519-25.
- [55]Sahari S, Saraswathy M. Syringic Acid Alleviates Hyperglycemia by Regulating Hepatic Key Enzymes of Carbohydrate Metabolism in Streptozotocin-induced Diabetic Rats. Asian Journal of Biological and Life Sciences. 2023;12(1):67.
- [56]Bouyahya A, El Omari N, et al. Moroccan antidiabetic medicinal plants: Ethnobotanical studies, phytochemical bioactive compounds, preclinical investigations, toxicological validations and clinical evidence; challenges, guidance and perspectives for future management of diabetes worldwide. Trends in Food Science & Technology, 2021;115:147-254.
- [57]Yoon SY, Ahn D, et al. Linoleic acid exerts antidiabetic effects by inhibiting protein tyrosine phosphatases associated with insulin resistance. Journal of Functional Foods. 2021;83:104532.
- [58]López-Gómez C, Santiago-Fernández C, et al. Oleic acid protects against insulin resistance by regulating the genes related to the PI3K signaling pathway. Journal of Clinical Medicine. 2020;9(8):2615.