Association of gut microbiota in the pathogenesis of acute respiratory distress syndrome and possible pharmacotherapy by sphingosine-1-phosphate
Abstract
The acute respiratory distress syndrome (ARDS) is one of the major heterogeneous clinical manifestations of severe respiratory failure developing in response to pathogenetic mechanisms and several inflammatory insults. The translocation of gut microbiota has a crucial impact on the pathogenesis of ARDS. Hence, a deeper understanding of the interplay of the gut microbiota would allow shedding valuable insights into both the pathogenesis of ARDS and the development of effective therapeutic interventions. Moreover, the modulation of gut-lung axis could also play a pivotal role in minimizing the lung dysbiosis with gut microbiota. There is little question that greater study of the gut-lung axis in critically ill patients is required to establish causal links between the shifted microbiota, infections, inflammation, and acute lung damage. It is worth mentioning that the lack of effective preventative measures is one of the main reasons for the increased mortality rate of 30-40% in ARDS patients. Some antibiotics and nanosized drugs showed positive results in ARDS management to some extent in pre-clinical or even first stages of clinical trials but large-scale results had been controversial. However, sphingosine-1-phosphate (S1P) showed hopeful results in ARDS patients by facilitating both systemic and endothelial integrity. Numerous investigations have shown the immunological connection between the gastrointestinal tract and the respiratory system. As the SphKs /S1P /S1PL metabolic pathway is associated with a wide variety of human illnesses (including respiratory diseases), it should come as no surprise that influencing intracellular S1P levels would have therapeutic promise in reducing the severity of lung diseases like ARDS.
INTRODUCTION
Acute respiratory distress syndrome (ARDS) is a type of pulmonary oedema which is non-cardiogenic and caused by an alveolar injury that is secondary to inflammatory processes which could either be systemic or pulmonary in origin [1]. Considering from a microscopic level, ARDS is correlated with diffuse alveolar damage and capillary endothelial injury [2]. Unfortunately, the syndrome remains extremely prevalent, and more than 100,000 patients are affected every year in the United States and only 34% of them are discharged home after surviving [3].
In terms of the development of ARDS, several pathophysiologic derangements are involved including increased epithelial and endothelial permeabilities as well as dysregulated inflammation [4]. The presence of gut microbiota plays a vital role in the pathogenesis of ARDS and Enterobacteriaceae as well as Bacteroidetes seem to be in a substantial number [5]. Studies have shown the translocation and enrichment of gut-microbiota in the lung of 91 ARDS patients on ventilator [6]. Furthermore, lung dysbiosis with gut microbiota could be responsible for immunosuppression and lung dysfunction leading to ventilator-associated pneumonia [7]. It is worth mentioning that the gut microbiota plays a major role by serving as a critical marker of ARDS pathogenesis [8].
High-throughput analysis of microbial 16S ribosomal RNA genes has shown the presence of microorganisms in lung tissue, challenging the long-held notion that the lungs are germ-free [9]. Similarly, to the gut microbiome, the lung microbiome is thought to have a role in host immunity via immunological priming, and an unbalanced environment in the lungs may increase the risk of developing respiratory illness [10, 11]. The immunological connection between the digestive tract and the lungs has been the subject of an increasing number of investigations [12, 13]. Many studies have looked at how gut microbiota might affect lung immunity, and some have shown a correlation between gut microbiome shifts and lung immunity [14, 15].
Despite the fact that numerous potential risk factors for ARDS are known, the condition cannot be reduced via preventative measures [2]. Moreover, the majority of the extensive phase 2 and 3 trials of therapeutic approaches in ARDS have failed to establish effectiveness, despite promising preclinical and early clinical results [1]. Disease-specific interventions are carried out in managing ARDS [16] and positive outcomes had been reported with the administration of different antibiotics. It includes but is not limited to ceftazidime [17], levofloxacin [18], colistin [19], vancomycin [20] and tobramycin [21]. The development and administration of nanosized drugs in ARDS management are also focused on due to positive pre-clinical results; nevertheless, several issues are yet to be addressed before clinical translation [22].
Sphingosine-1-phosphate (S1P) showed promising outcomes in ARDS patients. They mainly target the molecules stabilizing epithelial and endothelial cell-cell junctions, given the central role of epithelial and endothelial permeabilities in the pathogenesis of ARDS [4]. S1P is a lipid and G protein-coupled receptors on the endothelial cells mediating the integrity of the endothelial barrier [23]. Moreover, sphingosine-1-Phosphate facilitates both systemic and endothelial integrity as per different in vivo and in vitro models [24]. In addition to providing insight into the processes behind endothelium and epithelial permeabilities, researching proteins that assist maintain the endothelial and epithelial barriers offers therapeutic promise.
TRANSLOCATION OF GUT MICROBIOTA
Even though the presence of bacteria in alveoli is quite low in numbers, however, they play a vital role in maintaining immune homeostasis in the lung [25]. The typical bacterial variability of the lung microbiome is severely disturbed in critically sick patients, leading to the replacement of commensals by pathogens mostly coming from the skin and gut [26]. The translocation of the microbiome is highly interconnected with the elevated markers of lung injury and adverse inflammation [27].
In both infective and non-infective chronic pulmonary diseases, the composition of lung microbiota had been scrutinized [28]. In acute pulmonary diseases like ARDS, the presence of gut microbiota is elevated in the lung at the presence of Enterobacteriaceae and Bacteroidetes which refers to the phenomenon called ‘more gut in the lung’ [5]. The gut is susceptible to hyper-permeability in acute situations where the translocation of bacteria through the colon walls reaches the lung causing lung inflammation, infection and acute pulmonary damage [29]. The elevated permeability of the gut is also associated with an elevated alveolar-capillary hyper-permeability [6].
The patients requiring invasive mechanical ventilation because of respiratory failure seem to have the pathogenic inoculum originating from the oral flora, and their composition is gradually changed during ICU stay [30]. Hosts with increased susceptibility could end up in developing respiratory infection because of the ceaseless micro-aspiration of potential pathogens [31]. Research on ARDS has shown that, specific gut microbe has the ability in markedly translocating across the bowel wall and entering the lungs [6].
Increased alveolar and gut permeability are believed to facilitate the entry of pathogens to lungs [32]. Even though the complicated interconnectedness between the lung and gut microbiota are not properly understood, studies have indicated that the unsettled lung-gut axis could have a major impact on the pathogenesis of pulmonary impediment especially among the patients who are critically ill [33, 34].
A substantial change has been observed in the lung microbiome among patients who are critically ill [35]. Moreover, a decreased bacterial activity as well as a single population could be seen among the patients receiving mechanical ventilation alongside an increased presence of opportunistic pathogens that have the potential of becoming dormant [36] Many risk factors for ARDS contribute to this microbial dysbiosis by altering the metabolic (nutrient presence) and physiochemical (free radicals, pH, oxygen tension) environments of the alveoli [37].
One research study has shown that due to the pathogenic links between the lung and gut of critically ill patients, the lung microbiome could be altered [38]. Even though there has been a definite lack of data, researchers’ have proposed that the bacterial migration route could be responsible for systemic circulation or gut draining lymphatics [39]. Thus, it is important to determine whether the pathogens that are present in the lung of the patients, especially the ones susceptible to ARDS, could have a negative impact on them [38].
Though intestinal microflora is not susceptible to change in healthy individuals, specific health conditions could have the ability to create an imbalance in gut microflora including cancer [40] diabetes [41] as well as patients with obesity [42]. The intestinal barrier is one of the main reasons for both the translocation of bacteria and endotoxin resulting in sepsis that causes lung injury [43], which is one of the major initiating factors of ARDS [43]. However, the process by which the intestine alters the microflora and mucosal barrier during ARDS is not well comprehended [43]. In a Rat ARDS model, a close relationship was observed between ARDS and microflora where ARDS was induced due to the activation of the systemic inflammatory response, hypoxemia, damage by free radicals, injury to inflammatory mediators, immune irritation and destruction of intestinal mucosa barrier that eventually changed the intestinal environment [43]. There is a strong correlation between the severity of ARDS and systemic inflammatory reactions [44]. Additionally, patients with systemic inflammatory response syndrome have shown lower numbers of Lactobacillus and Bifidobacterium with a higher number of Pseudomonas and Staphylococcus [36].
ROLE OF GUT MICROBIOTA AND LUNGS IN HEALTH AND ARDS
Both the gut and the lung have many common characteristics, and the way in which these organs communicate with one another is influenced by both internal and external factors [45]. The composition of the gut microbiota determines whether or not the host will be healthy and able to fight off infections. Emerging research suggests the gut microbiota may influence pulmonary health and illness through cross talk with the lungs [45]. Various kinds of microbiota populate the lungs after being exposed to environmental stimuli and communication between the microbiota in the gut and those in the lungs could play a significant role in the development of some chronic lung disorders [46]. It is worth mentioning that modulation of the lung microbiota relies on both local inter-kingdom interaction and on crosstalk between the gut and lung [47].
As the field of microbiology has progressed, more and more data has surfaced suggesting that the gut microbiota may be of great importance in maintaining host health by strengthening defenses against enteral pathogens and maintaining immunological homeostasis [48]. Alterations in immunological responses and respiratory homeostasis have been linked to changes in the composition of the gut microbiota [49]. Some epidemiological and experimental research has shed light on the importance of cross talk between gut microbiota and lungs, a relationship coined the "gut-lung axis," although the mechanisms and pathways underlying this relationship remain unclear [46].
Recent research on sepsis and ARDS suggests that gut-associated bacteria may colonize the lungs [5]. It has been hypothesized that, under these circumstances, bacteria from the digestive tract would be able to translocate into the pulmonary circulation and this process is facilitated by increased gut and alveolo-capillary permeability [5].
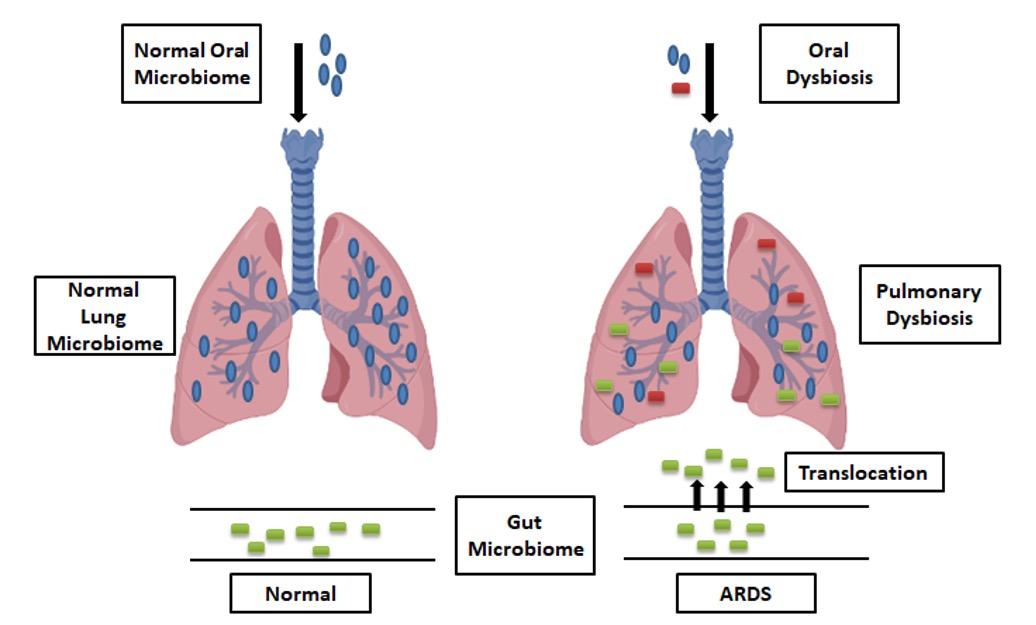
Figure 1 depicts that microbiomes found in a healthy person's lungs and intestines are quite distinct. Lung microbiome composition is quite similar to that of the oropharynx. Lung microbiome could get saturated with gut-associated microorganisms in severely sick individuals with sepsis, severe trauma, or ARDS. The aspiration and translocation of the potential pathogens from the gastrointestinal tract could give rise to infection in the lungs. As a consequence, dysbiosis is fostered, which in turn encourages inflammation and severe lung damage [5].
In critically sick ARDS patients, there may be pathogenic linkages between the gut and the lungs, and recent research shows that these interactions may affect the lung microbiome as well [33, 38]. A culture-dependent 16s rRNA gene sequencing method was used by Dickson and colleagues, and it was discovered that the lung Microbiota in sepsis mouse model and the patients with established ARDS became populated with gut bacteria where Bacteroides had been dominating in both the settings [50].
PATHOGENICITY OF ARDS RELATED TO GUT MICROBIOTA
There has been a lot of research done on how the gut microbiota influences the immune system [51]. In a nutshell, the gut microbiota uses both pro-inflammatory and regulatory signals to engage extensively with the mucosal immune system [52]. The capacity of neutrophils to extravasate from the blood is likewise affected by this [53]. It is now understood that gut microbiota has far-reaching immunological effects, particularly on the pulmonary immune system, beyond the local immune control by the site-specific bacteria [54]. Translocation of complete bacteria, their pieces, or metabolites past the intestinal barrier, into the systemic circulation, and modulating the lung immune response is possible through the mesenteric lymphatic system, a critical link between the lungs and the gut [55, 56, 57]. The gut-lung axis is an ultimate outcome of the intricate interactions between the microbes that make up the gut and lung microbiotas, as well as the local and systemic immunological responses that come from these interactions [47]. According to recent research using a mouse model, all these interactions clearly point to the gut-lung axis playing a significant role in respiratory illnesses [58].
Microbes from the gastrointestinal tract are abundant in the lower respiratory tract in ARDS patients with sepsis [50], indicating connections between the lower respiratory tract and gastrointestinal tract [59]. In the BAL samples of sepsis and ARDS patients, a major alteration of lung microbiota was seen where the enrichment of gut-specific microbes was confirmed correlating with alveolar tumor necrosis factors-α that provides proof of the translocation of gut and lung microbiota in critically ill patients [60]. Moreover, another study confirmed that the enrichment of Enterobacteriaceae and smoking-related taxa like Prevotella and Fusobacterium was seen in critically sick individuals who developed ARDS [33]. Consistently, a study by Kyo and colleagues has found that the presence of Staphylococcus, Streptococcus, and Enterobacteriaceae in BAL samples from 47 mechanically ventilated patients with or without ARDS was strongly linked with serum IL-6 and mortality [59].
Both in the preclinical models of established ARDS and sepsis, the lung microbiota had a major impact in the pathogenesis of the disease [61]. Furthermore, in a recent study, an oral commensal called Mycoplasma salivarium was enriched in lower airway of COVID-19 patients and it was shown to be associated with worse clinical outcomes especially for the ones who were undergoing mechanical ventilation [62]. Additionally, recent research with over 300 patients on mechanical ventilation showed that Staphylococcus or Pseudomonadaceae enrichment of the lower airways had been related to higher lower airway inflammation as well as diminished clinical outcomes that includes longer time require to liberate from mechanical ventilation and poorer 30-day survival [63]. Collectively, these results implicate microbial dysbiosis as a potential mediator of the observed association between dysregulated local and systemic inflammation and worse clinical outcomes in acute respiratory failure [61].
MODULATION OF GUT-LUNG AXIS: POTENTIAL TREATMENT TARGET FOR ARDS
The gut-lung axis immunological responses are especially dependent on the diversity and stability of the gut microbiota [64]. The regulated correlation between the antigens and metabolites of symbiotic microbiota with the host is of great importance as it activates the pattern recognition receptors, metabolic sensor receptors including G-protein coupled receptors as well as the sufficient production of inflammatory mediators; all these are necessary for the migration, activation and proliferation of both the innate and adaptive immune cells as they produce pro inflammatory cytokines, anti-inflammatory cytokines, antimicrobial peptides and immunoglobulins as well [65]. Through the circulatory and lymphatic systems, these cells and chemicals may go back and forth between the lungs and the intestinal tract, where they help control immunological and inflammatory responses [66].
Lung disorders caused by bacteria have a promising new treatment option which is the modification of the gut microbiota, mostly using probiotics [64]. Lung modifications draw attention to probiotic species from the Lactobacillus genus, despite the well-documented fact that various probiotic species and strains function differently depending on their metabolic pathways as well as interactions with the host [64]. A major positive activity of lung macrophages, less tissue inflammation and diminished lung bacterial load was associated with the increased level of IL-4, IL-10, enhanced production of IgG and IgA, increased frequency of T-reg cells, decreased TNF-α and IL-6 levels [64]. It highly states a significant resolving anti-inflammatory profile after the modulation of the intestinal microbiota of the host.
It is worth mentioning that the microbiome of the respiratory tract is dynamic and constantly changing. Microbial immigration via inhalation and micro aspiration as well as microbial removal through coughing, mucociliary transport, and immunological responses are the main processes that govern the balance of the microbiota [61]. Researchers hypothesize that eubiosis in the lower respiratory tract may be influenced by variables like as vaginal delivery [67], physical activity, and a balanced diet, whereas obesity, a sedentary lifestyle, and frequent consumption of antibiotics may have a negative effect on the microbiome of the lower airway.
CURRENT THERAPEUTIC OPTIONS
Several pharmacologic therapies have been assessed in both the second and third phases of clinical trials in treating ARDS [68]. With support from the National Heart, Lung and Blood Institute, clinical trials were carried out in exploring the effects of inhaled nitric oxide [69], and glucocorticoids [70], with a view to minimizing the negative impact of lung injury and enhancing supportive care which would ensure more days without the usage of ventilator [68, 71]. That being said, patients affected with ARDS might need mechanical ventilation though it could damage the lungs leading to the progression of a more severe form of ARDS called ventilator-associated lung injury (VALI) [72].
Thus, there had been a major focus on the development of nanosized drug delivery system targeting the lung in exploiting the distinct features of inflammatory microenvironments like elevated temperature, low pH conditions and particular enzyme-rich environment [73]. Even though some positive and promising results have been obtained from pre-clinical studies, before clinical translation, nanoparticles pose great barriers that are required to be taken into account [74]. They are mostly associated with nanoparticle engineering challenges, biological challenges, regulatory challenges and process challenges [75]. In any case, it's important to note that the advancement of nanotechnology has opened up new possibilities for the creation of novel and effective therapeutic intervention in treating ARDS, with the potential to utilize targeting mainly the macrophage-specific inflammatory pathways, while delivering diagnostic cargo to the activated macrophages [75]. Some of the major nano-systems that are used in pre-clinical stage include GNPs [76], nano emulsion [77], lipid-core nano capsules [78], unilamellar liposomes [79], cerium oxide NPs [80], mannan-coated liposomes [81] and PLGA-NPs [82] as well. The findings from large scale clinical studies could be beneficial in facilitating future research on the effective application of nanomedicine in addressing ARDS [75].
However, disease specific and complication-specific therapy is the mainstay of ARDS management, after accurate diagnosis of the underlying condition [16]. Thus, positive health outcomes could be achieved in ARDS patients with the usage of appropriate source control and antibiotics [83]. It might include Imipenem [84], Vancomycin [85], and Fosfomycin [86] as well. Like being said earlier, one of the most used drugs in treating ARDS is glucocorticoids as it improves both the airway pressure and oxygenation [16].
ROLE OF SPHINGOSINE-1-PHOSPHATE IN REDUCING MORTALITY IN ARDS
Sphingolipids play a vital role in a wide range of pulmonary disorders including acute lung injury (ALI) and ARDS [87]. They are one of the essential constituents of plasma membranes regulating some pathophysiological cellular responses inducing mast cell activation, apoptosis and cell survival, airway smooth muscle function and vascular permeability [88].
A protective effect is exerted by S1P against ALI [87]. S1P is considered a major angiogenic factor that helps preserve the barrier function and endothelial integrity of human lungs [89] as it prevents the alveolar flooding that has been demonstrated in ARDS animal models. S1P is mainly a bioactive sphingolipid acting both intracellularly and extracellularly (through S1P1-5; its G protein-coupled receptors) as shown in Figure 2.
However, it is worth mentioning that Sphingosine kinases 1 and 2 phosphorylate the sphingosine that generates the intracellular S1P [90]. It can also act as an intracellular messenger in the cell or the S1P transporters spinster homologue 2 (Spns2) can assist them in transporting outside of the cells [91]. The extracellular S1P can bind to the receptors coupled with five G-proteins and activate the signaling pathways that are associated with the cell proliferation, survival, migration and vascular integrity [92, 93].
The vascular barrier function is enhanced by S1P by a number of cellular events that are initiated by the activation of the G-protein coupled receptor- S1P1, as well as the downstream activation of Rac1 and Rho (intracellular signals), eventually resulting in endothelial cytoskeletal reorganization in forming stronger cortical rings of actin in the periphery of the cells that help to prevent the excessive and abnormal vascular permeability [94]. The vascular permeability is reduced by stimulating the S1P-SIP1 axis, which is proven to add great value in treating ARDS [87]. Alongside S1Ps, the S1P analogs (ftysiponate and FTY720) have demonstrated their efficacy being protective components in reducing lung oedema formation, attenuating parenchymal accumulation of some inflammatory cells which have the ability in limiting the progression of ARDS [95].
The role of S1P as one of the major regulators of endothelial barrier function is highly associated with the signaling via S1P1 and S1P2. It is important in activating the downstream Rho GTPases while rearranging the cytoskeleton as well [96]. The lung vascular permeability and inflammation caused by LPS have been found to be decreased in mouse models after intravenous infusion of S1P [97]. In most cases, researchers believe that ligation to S1P1 is responsible for S1P's barrier-enhancing actions that allows initiating a wide range of downstream signaling cascade such as cortactin translocation, Rac activation as well as rearranging the focal adherent’s junction proteins that culminate in increased lung endothelium cells barrier function [98, 99, 24].
Since S1P plays a barrier-protective function in ARDS, there has been progress in the development and application of S1PR (S1P receptor) agonists [100]. In HUVECs (human umbilical vein ECs), experiments show that FTY720 improves endothelial barrier function at low doses but disrupts the barrier and induces apoptosis at higher doses. The results of a mouse model of lung injury subjected to mechanical ventilation were similar [101]. Such a complex action of FTY720 in ECs is mainly because of the phosphorylation to FTY720-P by either SphK1 or SphK2 (two known isoenzymes in mammals [102] as they enhance the affinity for the S1PRs [103, 104]. Targeting S1PL (sphingosine-1-phosphate lyase) by using siRNA in HLMVECs (human lung microvascular endothelial cells) challenged with LPS, paralleled with murine studies pointed out declined secretion of IL-6, barrier disruption focusing that targeting S1PL might be a most important therapeutic option [105]. It is also worth mentioning that the recent discovery of innovative and more specialized pharmacological treatments in this field including ASMase inhibitors [106], S1P1 agonists [107], or SphK1 inhibitors [108], is reflective of the growing role of sphingolipids in respiratory illnesses.
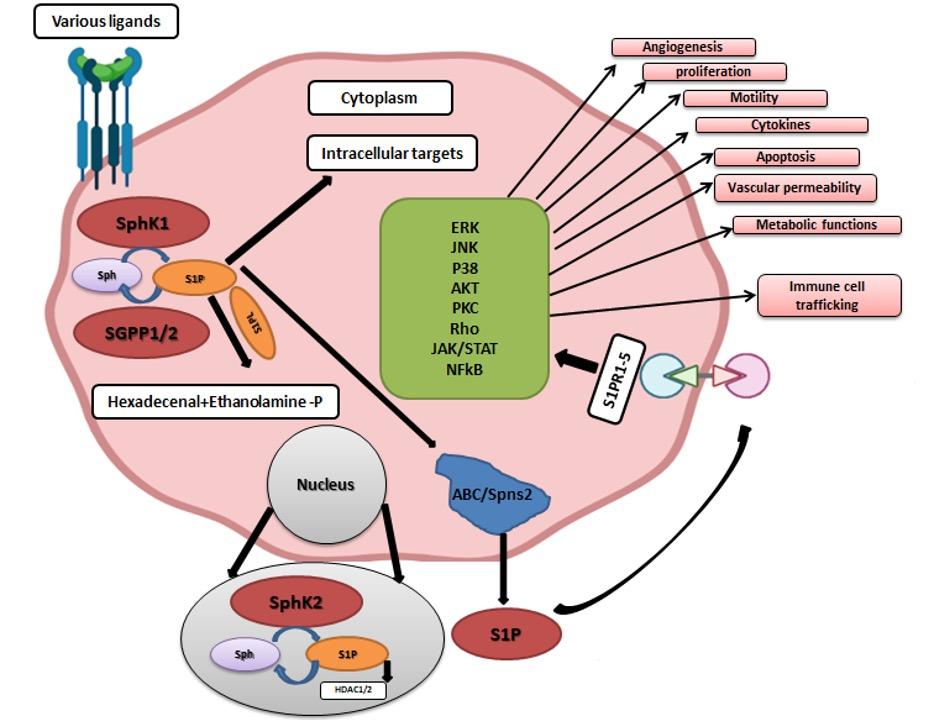
FUTURE PROSPECTS
The first description of ARDS was given by Ashbaugh and colleagues in 1967 [109]. Since then, numerous pharmacological interventions have targeted the principal pathophysiological mechanism of ARDS in clinical trials and almost all of them had shown negative results in terms of improving the patient outcome; nonetheless, the findings were promising in animal models [110]. It is worth mentioning that both antibiotics and nanosized drugs have been used in ARDS, but they did not bring substantial positive results on the patient outcome in terms of lowering morbidity and mortality in large trials [111], being controversial to be administered. Thus, the researchers have been investigating the pathogenesis of ARDS in order to get more insight as it is essential to understand both the causes and mechanisms of ARDS for effective new treatment development [112].
The study presented a greater insight into the pathogenesis of ARDS by shedding light on the details of the translocation of gut microbiota in the lung of ARDS patients. Such a phenomenon is crucial as a more detailed understanding is obtained regarding the role of gut microbiota is one of the most important and critical markers in the pathogenesis of ARDS [8]. The study would enable researchers to assess lung injury and adverse inflammation due to the translocation of gut microbiota [27]. Moreover, a clear understanding of the initiating factor of ARDS could also be obtained. These factors would play a vital role in the pharmacologic development in managing and reducing the death rate of ARDS.
Inflammatory cytokines and biomarkers of the injury of the cell happen to differ by ARDS clinical risk, proposing that the pathophysiology might vary by the associated clinical risk factors [113, 114]. In terms of clinical trials, most of the trials had recruited a heterogeneous cohort of ARDS patients while not taking into account the etiology [111]. It could be one of the reasons for the negative outcome of the pharmacological interventions. In order to do so, developing an understanding of the underlying mechanisms and pathogenesis of ARDS would be required which has been the focus of the study. Moreover, the timely detection of gut microbiota in ARDS could improve patient outcomes [5]. The study indicates that further investigation would be required regarding the process of bacterial translocation to the lung during ARDS as timely and precise bacterial identification and characterization could be helpful in administering the appropriate therapeutic intervention to manage the complication. The testing of new therapies to suppress this potentially crucial pathogenic process may need the usage of radio-imaging tools to observe bacterial translocation to the lung [115].
Major difficulties arise in the development of effective treatment strategies due to the interconnected nature of dysbiosis, inflammation, infections, and tissue damage that defines the disorder [38]. Thus, the most effective therapeutic interventions are administered. The study shows the positive role of S1P in the management of ARDS. S1P had been able to demonstrate positive results by facilitating both systemic and endothelial integrity in ARDS patients. They are also essential for preserving the barrier function in human lungs acting both intracellularly and extracellularly [89]. A detailed overview of this bioactive sphingolipid would enable researchers to dive deeper into the processes behind endothelial and epithelial permeabilities for offering the development of novel and effective therapeutic interventions in ARDS management. With more research on ARDS, it would be possible to develop a promising treatment for ARDS while unveiling the undiscovered and unexplored pathogenesis of ARDS.
CONCLUSION
ARDS has been one of the major concerns for both researchers and clinicians over the last 50 years even though promising pre-clinical data on therapeutic interventions are being obtained. But the data seem to have failed in demonstrating the efficacy in large phase 2 and phase 3 studies. However, sphinsosine-1-Phosphate; being an angiogenic factor, had shown some positive outcomes by preserving the barrier function of the human lung. On top of that, the association between gut microbiota in ARDS pathogenesis could give researchers a broad overview in developing effective therapeutics as the translation of gut microbiota plays a major role in the pathophysiological changes in the lung and the development of ARDS. Thus, we believe that a proper understanding of the lung microbiome, dysbiosis and translocation of the gut microbiome to the lung might help in the prevention and advancement of effective treatment of ARDS.
ACKNOWLEDGEMENTS
A special thanks to the corresponding author Mr. Fahd Bin Zahed for supervising the manuscript. His active involvement and remarkable assistance in nurturing the idea and monitoring the progress substantially enhanced the quality of the manuscript.
AUTHORS CONTRIBUTION
FBZ and TA conceived the idea and prepared the outline of the review. FBZ and TA performed the literature search and data extraction, analysis of extracted data and manuscript preparation. FBZ supervised the manuscript preparation and prepared the final draft. The authors read and accepted the final version of the manuscript.
CONFLICTS OF INTEREST
There is no conflict of interest among the authors.
References
- [1]Sweeney RM, McAuley DF. Acute respiratory distress syndrome. The Lancet. 2016; 388(10058):2416-2430.
- [2]Meyer NJ, Gattinoni L. et al. Acute respiratory distress syndrome. Lancet. 2021; 398(10300):622-637.
- [3]Rubenfeld GD, Caldwell E, et al. Incidence and outcomes of acute lung injury. The New England journal of medicine. 2005; 353(16):1685-1693.
- [4]Huppert L, Matthay M, et al. Pathogenesis of Acute Respiratory Distress Syndrome. Seminars in Respiratory and Critical Care Medicine. 2019;40(01):031-039.
- [5]Mukherjee S, Hanidziar D. More of the Gut in the Lung: How Two Microbiomes Meet in ARDS. The Yale Journal of Biology and Medicine. 2018;91(2):143-149.
- [6]Dickson RP, Singer BH, et al. Enrichment of the lung microbiome with gut bacteria in sepsis and the acute respiratory distress syndrome. Nature Microbiology. 2016;1(10).
- [7]Fromentin M, Ricard JD, et al. Respiratory microbiome in mechanically ventilated patients: a narrative review. Intensive Care Medicine. 2021;47(3):292-306.
- [8]Gopal G, Muralidar S, et al. Microbiome in Acute Respiratory Distress Syndrome (ARDS). Microbiome in Inflammatory Lung Diseases. Published online. 2022:117-134.
- [9]Lee YT, Kim KH, Hwang HS, et al. Innate and adaptive cellular phenotypes contributing to pulmonary disease in mice after respiratory syncytial virus immunization and infection. Virology. 2015; 485:36–46.
- [10]Beck JM, Young VB, et al. The Microbiome of the Lung. Translational Research: The Journal of Laboratory and Clinical Medicine. 2012; 160:258–66.
- [11]de Steenhuijsen Piters WAA, Sanders EAM, et al. The role of the local microbial ecosystem in respiratory health and disease. Philosophical Transactions of the Royal Society B: Biological Sciences. 2015;370.
- [12]Barfod K, Roggenbuck M, et al. The murine lung microbiome in relation to the intestinal and vaginal bacterial communities. BMC Microbiology. 2013; 13:303.
- [13]Segal LN, Blaser MJ. A Brave New World: The Lung Microbiota in an Era of Change. Annals of the American Thoracic Society. 2014; 11:S21–7.
- [14]Russell SL, Gold MJ, et al. Perinatal antibiotic treatment affects murine microbiota, immune responses and allergic asthma. Gut Microbes. 2013; 4:158–64.
- [15]Michael, Zahs A, et al. An alteration of the gut-liver axis drives pulmonary inflammation after intoxication and burn injury in mice. 2014; 307:G711–8.
- [16]Araz O. Current Pharmacological Approach to ARDS: The Place of Bosentan. The Eurasian Journal of Medicine. 2020; 52:81–5.
- [16]Araz O. Current Pharmacological Approach to ARDS: The Place of Bosentan. The Eurasian Journal of Medicine. 2020; 52:81–5.
- [17]Lu Q, Yang J, et al. Nebulized Ceftazidime and Amikacin in Ventilator-associated Pneumonia Caused by Pseudomonas aeruginosa. American Journal of Respiratory and Critical Care Medicine. 2011; 184:106–15.
- [17]Lu Q, Yang J, et al. Nebulized Ceftazidime and Amikacin in Ventilator-associated Pneumonia Caused by Pseudomonas aeruginosa. American Journal of Respiratory and Critical Care Medicine. 2011; 184:106–15.
- [18]Geller DE, Flume PA, et al. Levofloxacin Inhalation Solution (MP-376) in Patients with Cystic Fibrosis with Pseudomonas aeruginosa. American Journal of Respiratory and Critical Care Medicine. 2011; 183:1510–6.
- [18]Geller DE, Flume PA, et al. Levofloxacin Inhalation Solution (MP-376) in Patients with Cystic Fibrosis with Pseudomonas aeruginosa. American Journal of Respiratory and Critical Care Medicine. 2011; 183:1510–6.
- [19]Rouby JJ, Sole-Lleonart C, et al. Ventilator-associated pneumonia caused by multidrug-resistant Gramnegative bacteria: understanding nebulization of aminoglycosides and colistin. Intensive Care Medicine. 2020;46:766–70.
- [19]Rouby JJ, Sole-Lleonart C, et al. Ventilator-associated pneumonia caused by multidrug-resistant Gramnegative bacteria: understanding nebulization of aminoglycosides and colistin. Intensive Care Medicine. 2020;46:766–70.
- [20]Palmer LB, Smaldone GC. Reduction of Bacterial Resistance with Inhaled Antibiotics in the Intensive Care Unit. American Journal of Respiratory and Critical Care Medicine. 2014; 189:1225–33.
- [20]Palmer LB, Smaldone GC. Reduction of Bacterial Resistance with Inhaled Antibiotics in the Intensive Care Unit. American Journal of Respiratory and Critical Care Medicine. 2014; 189:1225–33.
- [21]Hallal A, Cohn SM, et al. Aerosolized Tobramycin in The Treatment of Ventilator-Associated Pneumonia: A Pilot Study. Surgical Infections. 2007; 8:73–82.
- [22]Hofmann-Amtenbrink M, Grainger DW, et al. Nanoparticles in medicine: Current challenges facing inorganic nanoparticle toxicity assessments and standardizations. Nanomedicine: Nanotechnology, Biology and Medicine. 2015; 11:1689–94.
- [23]Lee MJ, Thangada S, et al. Vascular Endothelial Cell Adherens Junction Assembly and Morphogenesis Induced by Sphingosine-1-Phosphate. Cell 1999; 99:301–12.
- [24]Xiong Y, Hla T. S1P Control of Endothelial Integrity. Current Topics in Microbiology and Immunology 2014; 378:85–105.
- [25]O’Dwyer DN, Dickson RP, et al. The Lung Microbiome, Immunity, and the Pathogenesis of Chronic Lung Disease. The Journal of Immunology. 2016;196(12):4839-4847.
- [26]Shukla SD, Budden KF, et al. Microbiome effects on immunity, health and disease in the lung. Clinical & Translational Immunology. 2017;6(3):e133.
- [27]Dickson RP. The microbiome and critical illness. The Lancet Respiratory Medicine. 2016;4(1):59-72.
- [28]Dumas A, Bernard L, et al. The role of the lung microbiota and the gut-lung axis in respiratory infectious diseases. Cellular Microbiology. 2018;20(12):e12966.
- [29]Fanos V, Pintus MC, et al. Lung microbiota in the acute respiratory disease: from coronavirus to metabolomics. Journal of Pediatric and Neonatal Individualized Medicine (JPNIM). 2020;9(1):e090139-e090139.
- [30]Perkins SD, Woeltje KF, et al. Endotracheal tube biofilm inoculation of oral flora and subsequent colonization of opportunistic pathogens. International Journal of Medical Microbiology. 2010;300(7):503-511.
- [31]Kalanuria A, Zai W, et al. Ventilator-associated pneumonia in the ICU. Critical Care. 2014;18(2):208.
- [32]Thompson BT, Chambers RC, et al. Acute respiratory distress syndrome. Drazen JM, ed. New England Journal of Medicine. 2017;377(6):562-572.
- [33]Panzer AR, Lynch SV, et al. Lung Microbiota Is Related to Smoking Status and to Development of Acute Respiratory Distress Syndrome in Critically Ill Trauma Patients. American Journal of Respiratory and Critical Care Medicine. 2018;197(5):621-631.
- [34]Samuelson DR, Welsh DA, et al. Regulation of lung immunity and host defense by the intestinal microbiota. Frontiers in Microbiology. 2015; 6:1085.
- [35]Kelly BJ, Imai I, et al. Composition and dynamics of the respiratory tract microbiome in intubated patients. Microbiome. 2016;4(1).
- [36]Yin Y, Hountras P, et al. The microbiome in mechanically ventilated patients. Current Opinion in Infectious Diseases. 2017;30(2):208-213.
- [37]Huffnagle GB, Dickson RP, et al. The respiratory tract microbiome and lung inflammation: a two-way street. Mucosal Immunology. 2016;10(2):299-306.
- [38]Dickson RP. The Lung Microbiome and ARDS. It Is Time to Broaden the Model. American Journal of Respiratory and Critical Care Medicine. 2018;197(5):549-551.
- [39]Deitch EA. Gut lymph and lymphatics: a source of factors leading to organ injury and dysfunction. Annals of the New York Academy of Sciences. 2010;1207:E103-E111.
- [40]Rana S. Importance of Methanogenic Flora in Intestinal Toxicity During 5-Fluorouracil Therapy for Colon Cancer. Journal of Clinical Gastroenterology. 2013;47(1):9-11.
- [41]Stachowicz N, Kiersztan A. The role of gut microbiota in the pathogenesis of obesity and diabetes. Postępy Higieny i Medycyny Doświadczalnej. 2013; 67:288-303.
- [42]Fei N, Zhao L. An opportunistic pathogen isolated from the gut of an obese human causes obesity in germfree mice. The ISME Journal. 2012;7(4):880-884.
- [43]Li Y. Changes in intestinal microflora in rats with acute respiratory distress syndrome. World Journal of Gastroenterology. 2014;20(19):5849.
- [44]Mustansir DF, Patel BK, et al. Gut Microbiota Dysbiosis as a Target for Improved Post-Surgical Outcomes and Improved Patient Care: A Review of Current Literature. Shock. 2020;55(4):441-454.
- [45]Zhou A, Lei Y, Tang L, et al. Gut microbiota: The emerging link to lung homeostasis and disease. Journal of Bacteriology 2020.
- [46]Budden KF, Gellatly SL, et al. Emerging pathogenic links between microbiota and the gut–lung axis. Nature Reviews Microbiology. 2016; 15:55–63.
- [47]Enaud R, Prevel R, et al. The Gut-Lung Axis in Health and Respiratory Diseases: A Place for Inter-Organ and Inter-Kingdom Crosstalks. Frontiers in Cellular and Infection Microbiology 2020;10.
- [48]Marsland BJ, Trompette A, et al. The Gut–Lung Axis in Respiratory Disease. Annals of the American Thoracic Society 2015; 12:S150–6.
- [49]Voynow JA, Hendricks-Muñoz KD. The Gut–Lung Axis and Pulmonary Responses to Ozone. American Journal of Respiratory Cell and Molecular Biology 2018; 59:281–2.
- [50]Dickson RP, Singer BH, et al. Enrichment of the lung microbiome with gut bacteria in sepsis and the acute respiratory distress syndrome. Nature Microbiology. 2016;1.
- [51]Elson CO, Alexander KL. Host-Microbiota Interactions in the Intestine. Digestive Diseases 2015; 33:131–6.
- [52]Skelly AN, Sato Y, et al. Mining the microbiota for microbial and metabolite-based immunotherapies. Nature Reviews Immunology 2019; 19(5):305-323.
- [53]Karmarkar D, Rock KL. Microbiota signalling through MyD88 is necessary for a systemic neutrophilic inflammatory response. Immunology 2013; 140:483–92.
- [54]Chiu L, Bazin T, et al. Protective Microbiota: From Localized to Long-Reaching Co-Immunity. Frontiers in Immunology 2017;8.
- [55]Bingula R, Filaire M, et al. Desired Turbulence? Gut-Lung Axis, Immunity, and Lung Cancer. Journal of Oncology 2017; 2017:5035371.
- [56]Trompette A, Gollwitzer ES, et al. Gut microbiota metabolism of dietary fiber influences allergic airway disease and hematopoiesis. Nature Medicine 2014; 20:159–66.
- [57]McAleer JP, Kolls JK. Contributions of the intestinal microbiome in lung immunity. European Journal of Immunology 2017; 48:39–49.
- [58]Skalski JH, Limon JJ, et al. Expansion of commensal fungus Wallemia mellicola in the gastrointestinal mycobiota enhances the severity of allergic airway disease in mice. PLOS Pathogens 2018;14:e1007260.
- [59]Kyo M, Nishioka K, et al. Unique patterns of lower respiratory tract microbiota are associated with inflammation and hospital mortality in acute respiratory distress syndrome. Respiratory Research. 2019;20.
- [60]Yi X, Gao J, Wang Z. The human lung microbiome—A hidden link between microbes and human health and diseases. IMeta 2022.
- [61]Natalini JG, Singh S, et al. The dynamic lung microbiome in health and disease. Nature Reviews Microbiology 2022.
- [62]Sulaiman I, Chung M, et al. Microbial signatures in the lower airways of mechanically ventilated COVID-19 patients associated with poor clinical outcome. Nature Microbiology 2021; 6:1245–58.
- [63]Kitsios GD, Yang H, et al. Respiratory Tract Dysbiosis Is Associated with Worse Outcomes in Mechanically Ventilated Patients 2020;202:1666–77.
- [64]Cruz CS, Ricci MF, et al. Gut Microbiota Modulation as a Potential Target for the Treatment of Lung Infections. Frontiers in Pharmacology 2021; 12:724033.
- [65]Fan Y, Pedersen O. Gut microbiota in human metabolic health and disease. Nature Reviews Microbiology 2020;19.
- [66]Dang AT, Marsland BJ. Microbes, metabolites, and the gut–lung axis. Mucosal Immunology 2019; 12:843–50.
- [67]Pattaroni C, Watzenboeck ML, et al. Early-Life Formation of the Microbial and Immunological Environment of the Human Airways. Cell Host & Microbe 2018; 24:857-865.e4.
- [68]Matthay MA, Zemans RL. The Acute Respiratory Distress Syndrome: Pathogenesis and Treatment. Annual Review of Pathology: Mechanisms of Disease. 2011;6(1):147-163.
- [69]Taylor RW. Low-Dose Inhaled Nitric Oxide in Patients With Acute Lung InjuryA Randomized Controlled Trial. JAMA. 2004;291(13):1603.
- [70]National Heart, Lung, and Blood Institute (NHLBI). Acute Respiratory Distress Syndrome Clinical Network (ARDSNet). clinicaltrials.gov. Published March 22, 2016.
- [71]Matthay MA, McAuley DF, et al. Clinical trials in acute respiratory distress syndrome: challenges and opportunities. The Lancet Respiratory Medicine. 2017;5(6):524-534.
- [72]Ragaller M, Richter T. Acute lung injury and acute respiratory distress syndrome. Journal of Emergencies, Trauma, and Shock. 2010;3(1):43.
- [73]Barton GM. A calculated response: control of inflammation by the innate immune system. Journal of Clinical Investigation. 2008;118(2):413-420.
- [74]Hofmann-Amtenbrink M, Grainger DW, et al. Nanoparticles in medicine: Current challenges facing inorganic nanoparticle toxicity assessments and standardizations. Nanomedicine: Nanotechnology, Biology and Medicine. 2015;11(7):1689-1694.
- [75]Vichare R, Janjic JM. Macrophage-Targeted Nanomedicines for ARDS/ALI: Promise and Potential. Inflammation. 2022; 45(6):2124-2141.
- [76]Gao W, Wang Y, et al. Size-dependent anti-inflammatory activity of a peptide-gold nanoparticle hybrid in vitro and in a mouse model of acute lung injury. Acta Biomaterialia. 2019; 85:203-217.
- [77]Wang Y, Ding L, et al. Treatment of acute lung injury and early- and late-stage pulmonary fibrosis with combination emulsion siRNA polyplexes. Journal of Controlled Release. 2019; 314:12-24.
- [78]de Oliveira MTP, de Sá Coutinho Éverton D, et al. Orally delivered resveratrol-loaded lipid-core nanocapsules ameliorate LPS-induced acute lung injury via the ERK and PI3K/Akt pathways. International Journal of Nanomedicine. 2019; 14:5215-5228.
- [79]Li N, Weng D, et al. Surfactant protein-A nanobody-conjugated liposomes loaded with methylprednisolone increase lung-targeting specificity and therapeutic effect for acute lung injury. Drug Delivery. 2017;24(1):1770- 1781.
- [80]Niemiec SM, Hilton SA, et al. Cerium oxide nanoparticle delivery of microRNA-146a for local treatment of acute lung injury. Nanomedicine: Nanotechnology, Biology and Medicine. 2021; 34:102388.
- [81]Wijagkanalan W, Higuchi Y, et al. Enhanced Anti-Inflammation of Inhaled Dexamethasone Palmitate Using Mannosylated Liposomes in an Endotoxin-Induced Lung Inflammation Model. Molecular Pharmacology. 2008;74(5):1183-1192.
- [82]Spence S, Greene MK, et al. Targeting Siglecs with a sialic acid–decorated nanoparticle abrogates inflammation. Science Translational Medicine. 2015;7(303):303ra140-303ra140.
- [83]Rhodes A, Evans LE, et al. Surviving Sepsis Campaign. Critical Care Medicine. 2017;45(3):486-552.
- [84]Radhakrishnan M, Jaganath A, et al. Nebulized imipenem to control nosocomial pneumonia caused by Pseudomonas aeruginosa. Journal of Critical Care. 2008;23(1):148-150.
- [85]Palmer LB, Smaldone GC, et al. Aerosolized antibiotics and ventilator-associated tracheobronchitis in the intensive care unit*. Critical Care Medicine. 2008;36(7):2008-2013.
- [86]Kollef MH, Ricard JD, et al. A Randomized Trial of the Amikacin Fosfomycin Inhalation System for the Adjunctive Therapy of Gram-Negative Ventilator-Associated Pneumonia. Chest. 2017;151(6):1239-1246.
- [87]Herrero R, Rojas Y, et al. Novel Pharmacologic Approaches for the Treatment of ARDS. Annual Update in Intensive Care and Emergency Medicine 2014. Published online 2014:231-243.
- [88]Uhlig S, Gulbins E. Sphingolipids in the Lungs. American Journal of Respiratory and Critical Care Medicine. 2008;178(11):1100-1114.
- [89]Abbasi T, Garcia JGN. Sphingolipids in Lung Endothelial Biology and Regulation of Vascular Integrity. Sphingolipids in Disease. Published online 2013:201-226.
- [90]Spiegel S, Milstien S. The outs and the ins of sphingosine-1-phosphate in immunity. Nature Reviews Immunology. 2011;11(6):403-415.
- [91]Kawahara A, Nishi T, et al. The Sphingolipid Transporter Spns2 Functions in Migration of Zebrafish Myocardial Precursors. Science. 2009;323(5913):524-527.
- [92]Maceyka M, Harikumar KB, et al. Sphingosine-1-phosphate signaling and its role in disease. Trends in Cell Biology. 2012;22(1):50-60.
- [93]Proia RL, Hla T. Emerging biology of sphingosine-1-phosphate: its role in pathogenesis and therapy. The Journal of Clinical Investigation. 2015;125(4):1379-1387.
- [94]Wang L, Dudek SM. Regulation of vascular permeability by sphingosine 1-phosphate. Microvascular Research. 2009;77(1):39-45.
- [95]Natarajan V, Dudek SM, et al. Sphingosine-1–Phosphate, FTY720, and Sphingosine-1–Phosphate Receptors in the Pathobiology of Acute Lung Injury. American Journal of Respiratory Cell and Molecular Biology. 2013;49(1):6-17.
- [96]Garcia JGN, Liu F, et al. Sphingosine 1-phosphate promotes endothelial cell barrier integrity by Edgdependent cytoskeletal rearrangement. Journal of Clinical Investigation 2001; 108:689–701.
- [97]McVerry BJ, Garcia JGN. Endothelial cell barrier regulation by sphingosine 1-phosphate. Journal of Cellular Biochemistry 2004; 92:1075–85.
- [98]Sun X, Shikata Y, et al. Enhanced interaction between focal adhesion and adherens junction proteins: Involvement in sphingosine 1-phosphate-induced endothelial barrier enhancement. Microvascular Research 2009; 77:304–13.
- [99]Yoh Takuwa, Okamoto Y, et al. Sphingosine-1-phosphate signaling in physiology and diseases 2012;38:329–37.
- [100]Ebenezer DL, Fu P, et al. Targeting sphingosine-1-phosphate signaling in lung diseases. Pharmacology & Therapeutics 2016; 168:143–57.
- [101]Holger SP, Müller, Hocke AC, et al. The Sphingosine-1 Phosphate receptor agonist FTY720 dose dependently affected endothelial integrity in vitro and aggravated ventilator-induced lung injury in mice 2011; 24:377–85.
- [102]Nava VE, Emanuela L, et al. Functional characterization of human sphingosine kinase-1 2000;473:81–4.
- [103]Billich A, Frédéric B, et al. Phosphorylation of the Immunomodulatory Drug FTY720 by Sphingosine Kinases 2003;278:47408–15.
- [104]Paugh SW, Payne SG, et al. The immunosuppressant FTY720 is phosphorylated by sphingosine kinase type 2 2003; 554:189–93.
- [105]Zhao Y, Gorshkova I, Evgeny Berdyshev, He D, Fu P, Ma W-L, et al. Protection of LPS-Induced Murine Acute Lung Injury by Sphingosine-1-Phosphate Lyase Suppression 2011;45:426–35.
- [106]Roth Anke G, Drescher D, et al. Potent and Selective Inhibition of Acid Sphingomyelinase by Bisphosphonates. Angewandte Chemie International Edition 2009; 48:7560–3.
- [107]Marsolais D, Yagi S, et al. Modulation of Chemokines and Allergic Airway Inflammation by Selective Local Sphingosine-1-phosphate Receptor 1 Agonism in Lungs 2010;79:61–8.
- [108]Puneet P, Yap CT, et al. SphK1 Regulates Proinflammatory Responses Associated with Endotoxin and Polymicrobial Sepsis. Science 2010; 328:1290–4.
- [109]Ashbaugh DG, Bigelow DB, et al. Acute respiratory distress in adults. Lancet (London, England). 1967;2(7511):319-323.
- [110]Bos LD, Martin-Loeches I, et al. ARDS: challenges in patient care and frontiers in research. European Respiratory Review. 2018;27(147):170107.
- [111]Boyle AJ, McNamee JJ, et al. Biological therapies in the acute respiratory distress syndrome. Expert Opinion on Biological Therapy. 2014;14(7):969-981.
- [112]Huppert L, Matthay M, et al. Pathogenesis of Acute Respiratory Distress Syndrome. Seminars in Respiratory and Critical Care Medicine. 2019;40(01):031-039.
- [113]Sheu CC, Gong MN, et al. Clinical Characteristics and Outcomes of Sepsis-Related vs Non-Sepsis-Related ARDS. Chest. 2010;138(3):559-567.
- [114]Calfee CS, Eisner MD, et al. Trauma-associated lung injury differs clinically and biologically from acute lung injury due to other clinical disorders. Critical care medicine. 2007;35(10):2243-2250.
- [115]Wang L, Llorente C, et al. Methods to determine intestinal permeability and bacterial translocation during liver disease. Journal of Immunological Methods. 2015; 421:44-53.