Harnessing plant–microorganism interactions for nano-bioremediation of heavy metals: Cutting-edge advances and mechanisms
Abstract
Nano-bioremediation, an emerging eco-friendly strategy that integrates nanotechnology and biological processes to mitigate the contamination of heavy metals from the environment. To explores synergistic interactions between plants and microorganisms, focusing on their potential role in enhancing nano-bioremediation are highly demandable. This study focuses several key mechanisms including biosorption, bioaccumulation, biomineralization, and enzymatic reduction, the coordination of microorganisms and plants in tolerating and transforming toxic heavy metals into less toxic forms. The potential role of microorganism-assisted nanomaterials, including nano-biosorbents and nano-catalysts in phyto- and eco-environments were updated. This review also highlights recent studies on the significance of plant-microbe systems and nanomaterials in heavy metal remediation, challenges such as microbial survival, scalability, and ecological impacts were addressed, alongside potential solutions. Finally, this critical review provides new insights into harnessing plant–microorganism interactions for nano-bioremediation, presenting an eco-friendly approach to address global heavy metal pollution, and it shows a sustainable way of clean environment.
INTRODUCTION
Bioremediation (BR) is one of the most efficient methods for removal of heavy-metal-from the contaminated soil and groundwater. This procedure is less harmful to the environment and more economical than conventional chemical and physical techniques, which are very costly and inefficient at low metal concentrations and result in large volumes of hazardous sludge [1,2]. The ability of microorganisms to breakdown contaminants is dependent on environmental conditions for growth and metabolism, which include favorable temperature, pH, and moisture [3,4]. Microorganisms are crucial for the removal of contaminants from soil, water, and sediments because of their benefits over alternative remediation techniques. These approaches of HMs removal are eco-friendly and cost effective. Additionally, they aid in the restoration of the natural ecosystem by preventing ongoing contamination [5]. Also, the contamination of heavy metals (HMs) has become a severe hazard to the ecosystem [6]. In the industrial sector, heavy metal is a profitable industry. Nevertheless, it is also a major environmental problem everywhere [7,8]. Also, the environment contains natural, agricultural, solid waste, inland effluents, and air sources, for the additional heavy metal sources. Large portions of the earth have been polluted by mining, electroplating, metallurgical smelting, pesticide, and fertilizer use in agricultural fields [9].
Furthermore, HMs that find their way into the environment linger and seriously endanger creatures that come into proximity to them due to toxicity. Although very low quality is crucial for the biological operations of plants and animals, but in high dose is toxic and inhibit metabolism in other organisms [10]. Toxic heavy metals, such as lead (Pb), cadmium (Cd), mercury (Hg), chromium (Cr), zinc (Zn), uranium (Ur), selenium (Se), silver (Ag), gold (Au), nickel (Ni), and arsenic (As), which are not useful to plants, inhibit plant growth, photosynthetic and enzymatic activities, and essential plant nutrition [11]. Moreover, even at low concentrations, heavy metals are carcinogenic to humans [12]. Conversely, bioremediators are biological agents used in bioremediation to help to clean contaminated sites. Among the most often used bioremediators are bacteria, archea, and fungi [13]. The application of bioremediation, a biotechnological method that uses microorganisms to solve and eliminate environmental concerns caused by different pollutants through biodegradation.
Scientists are coming to an agreement on how to reduce pollutant release and mitigate their impacts using living creatures such as plants, which is known as phytoremediation or bacteria, which is referred to as bioremediation [14]. To address these issues, biological methods such as biosorption, bioaccumulation, biodegradation, and bioremediation are used to remove heavy metal ions, providing an appealing alternative to physicochemical methods [15]. These methods are potentially simple, low cost, more effective, ecofriendly, and a self-sustaining option for wastewater amelioration, which is gaining new attention nowadays [16]. Also, the chemical methods for heavy metal remediation pose health hazards such as toxicity and environmental risks, coupled with limitations like high costs and inefficiency under varying conditions. In contrast, microorganism-assisted approaches offer eco-friendly, sustainable, and cost-effective alternatives, leveraging natural mechanisms for safe and effective HMs removal [2,17].
The goal of this review is to explore current trends in the application/role of microorganisms in bioremediation with their interactions for nano-bioremediation, find the necessary background information to fill in any gaps in this theme area. In this study, the nono-bioremediation strategy provides a sustainable way for removing or minimizing HMs from the plants, soils and environments.
SOURCES OF HEAVY METALS
Natural resources, such as air, soil, and water ecosystems, have been discovered to contain HMs, agricultural chemicals, industrial solvents (especially chlorinated solvents), and other types of pollution [18]. The use of HMs by industry and agricultural sectors has resulted in massive amounts of HMs being relinquished and disposed of inadvertently in most ecosystems [19]. Several agricultural practices, such as the irreversible use of urban sewage sludge, industry-based practices, such as composting and burning of garbage in a variety of techniques, and vehicle emissions unintentionally introduce toxic metals (Cd, Cr, Pb, Hg, As, Cu, Zn, and Ni) into soils (Figure 1). Motor vehicle emissions (Pb) [20], engine wear (Cd, Cu, and Ni) [21], and tire abrasion (e.g., Zn). According to Hunter [22], arsenic could have positive effects on gene silencing and methionine metabolism in animals. Arsenic and cadmium are naturally present in very small amounts in the Earth's crust and probably weren't conscripted during evolutionary processes because they are less abundant than P and Zn, which occupy adjacent columns in the periodic table, respectively [23].
The accumulation of potentially harmful quantities of As and Cd in soils is largely due to anthropogenic activity [24]. Metal toxicity is critical for living organisms, including microbes, plants, animals, and humans. However, the toxicity varies depending on the organism, while most of the 80 metals detected are essential for human functioning biology (e.g., Fe, Mg, Zn), others, such as Pb, Hg, and Cd, are among the oldest human toxicants [25]. A few heavy metals, including Fe, Cu, and Zn, are necessary microelements, whereas others, like Cd and Pb, have no beneficial function and are harmful even at low quantities (Figure 1). The contamination of soil and aquatic ecosystems is important, because metals are not biodegradable like other organic contaminants, they accumulate in terrestrial, aquatic, and marine ecosystems [26].
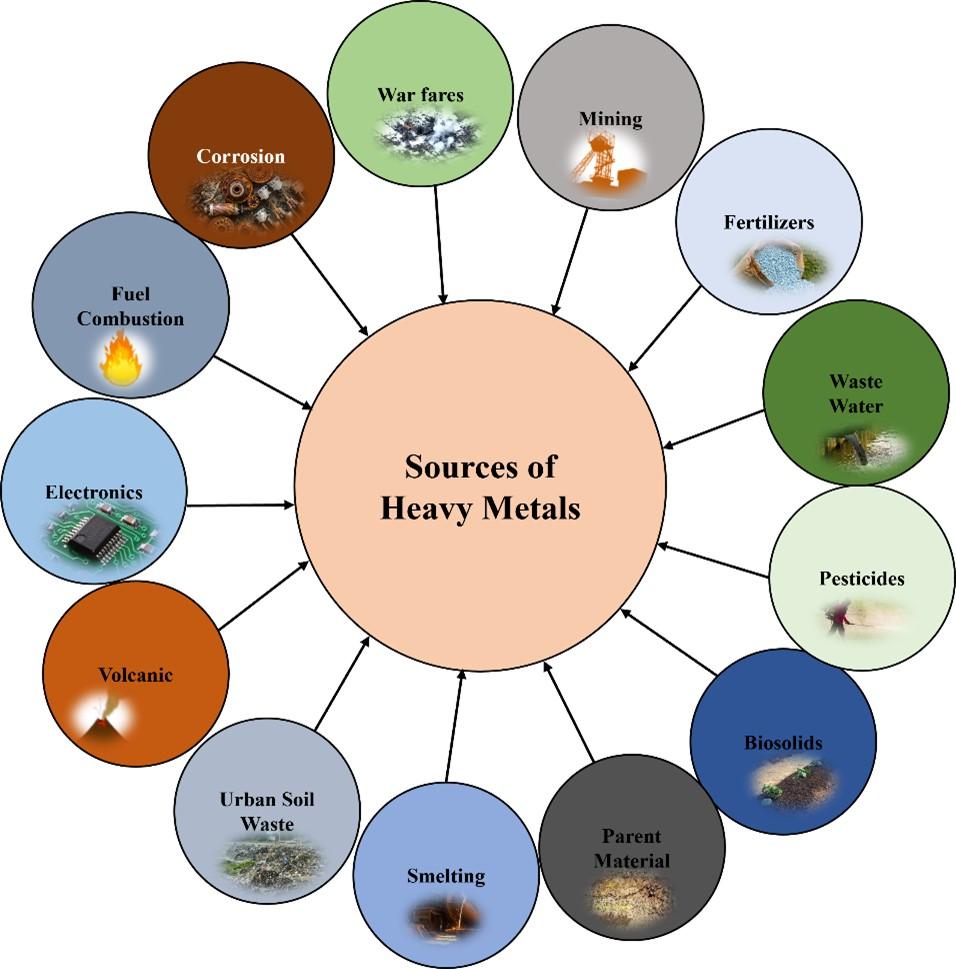
HEAVY METALS CONTAMINATION AND PUBLIC HEALTH RISK
HMs contamination problem is one of the major concerns worldwide. HMs toxicity varies due to their toxicity levels. The HMs are fluently added to environments from different sources (Figure 1), and those can easily deposit into living organisms [27]. They are all metals that have an atomic weight higher than that of iron (55.8 gmol–1), and they are found in the environment. However, some metals have an atomic weight lower than that of Fe. For example, Cr, and some metalloids, like As and Se, are also called "heavy metals" [28]. Many types of HMs can be micronutrients for humans. These include Cu, Fe, Mn, Mo, Zn, and Ni. They can also be toxic to humans if being exposed to them for a longer period, like Hg, Pb, Cd, Cu, Ni, and Co. Contamination by HMs has a lot of bad effects, not just on animals and plants but also on human health [29]. As an example, Zn is a component of a few enzymes, such as enzymes that break down carbohydrates, proteins, and peptides, as well as enzymes that make RNA and ribosomes in plants. Copper helps plants do a lot of things, like photosynthesis, respiration, carbohydrate distribution, nitrogen and cell wall metabolism, seed production, and disease resistance, but at high concentrations, these metals can harm cells [30]. The Cd is highly toxic for biological processes and very harmful to organisms when it builds up even in low quantities. Deposition of HMs depends on metal ions specific ion-binding processes to specific locations, and cellular structure variability. HMs ions, have a strong electrostatic attraction and great binding affinities with these same locations. The toxicity outputs of HMs are critical for basic genetic molecules of organisms. The structures and biomolecules, such as cell wall enzymes, DNA, and RNA, become unstable because of this, which is ultimately responsible for occurring mutations at molecular levels, as a result altering genetic levels, physiological difficulties, illnesses, and even cancer [31].
HEAVY METAL TOXICITY IN PLANTS, SOIL, AND ENVIRONMENT
Globally, due to the persistence, high toxicity, and recalcitrant nature, metal contamination has now become a serious concern in plants, soil, and the environment (Figure 2). These toxic metals have posed a serious threat to the environmental stability and health of all living organisms [2]. Like other living organisms, plants are not resistant to high concentrations of HMs in the air due to human activities and environment. Trace amounts of HMs enhance plants by acting as essential micronutrients. According to Lopez-Vargas et al. [32], copper can improve the flavor and color of floral arrangements, fruits, and vegetables by increasing sugar content in plants. Zn is an essential component of the enzymatic system as well as the metabolic processes of plants [33], and photosynthetic compounds are particularly influenced by HMs [34]. Heavy metals have been shown to accumulate in plants, where they interfere with the normal metabolic and biological processes in the plant, and eventually leading to severe yield losses [35].
HMs prevent seed germination by adversely influencing the processes, which in turn reduces the establishment of the entire stand [36]. Due to the high concentration of malondialdehyde (MDA) and H2O2, HM also impairs the water status of plants, damages the stability of their membranes, and increases the loss of crucial osmolytes. It shows that different chemical, physical, and biological methods for HMs removal from soils have been in practice globally. Plants consume and accumulate HM that is present in the soil at very high concentrations and eventually reaches human nutrition through the food chain [37]. Recently, microbes have gained a lot of attention from scientists worldwide. Biosorption, bioaccumulation, biovolatilization, biomineralization, oxidation and reduction, bioleaching, and the synthesis of bio-surfactants are some of the methods by which the bacteria extract the heavy metals from the soil [17].
Plants and microorganisms are used as biological methods to treat HMs containing polluted soils [38]. Hints, there are also certain drawbacks to these techniques in terms of long durations, environmental sensitivity, and toxicity of contaminants [39]. High levels of HMs in soil and water are representative examples of human activities which have a significant effect on the environment and present a huge risk [40,41]. According to Abd Elnabi et al. [42], living plants and animals are at serious risk due to the toxic HM's persistence in the soil environment. For terrestrial plants, the primary points of contact with hazardous heavy metals (HMs) are the roots [43]. Additionally, microbes lower the concentration of heavy metals (HMs) in soil. For example, Aspergillus niger shown a notable capacity to bioaccumulate Cd and Cr [44], and Stenotrophomonas rhizophila also considerably reduced Pb and Cu by 76.9% and 83.4%, respectively [45]. Microbes have a large surface area to adsorb the HMs because of their small size, which lowers the total amount of HMs available [46]. Soil biology is indispensable with respect to soil quality maintenance, which again is very important for sustainable agriculture. Human activities have emerged as the prime source of HMs and have disturbed the soil microbes, soil fertility, and productivity [47].
However, their bioaccumulation and biomagnification attributes in the food chain are highly threatening to the environment due to their accumulation in soil and plants [48]. Application of chemical fertilizers and pesticides can enhance the risk of HMs contamination in the soil. The chemical toxicity outputs lead to build-up in crop tissue grown in the contaminated soil [49]. Microbial bioremediation methods have recently demonstrated significant promise in cleaning up contaminated soils. One type of green technology is the utilization of microorganisms' metabolic processes to remove heavy metal contamination, and most HMs have been classified as hazardous overall.
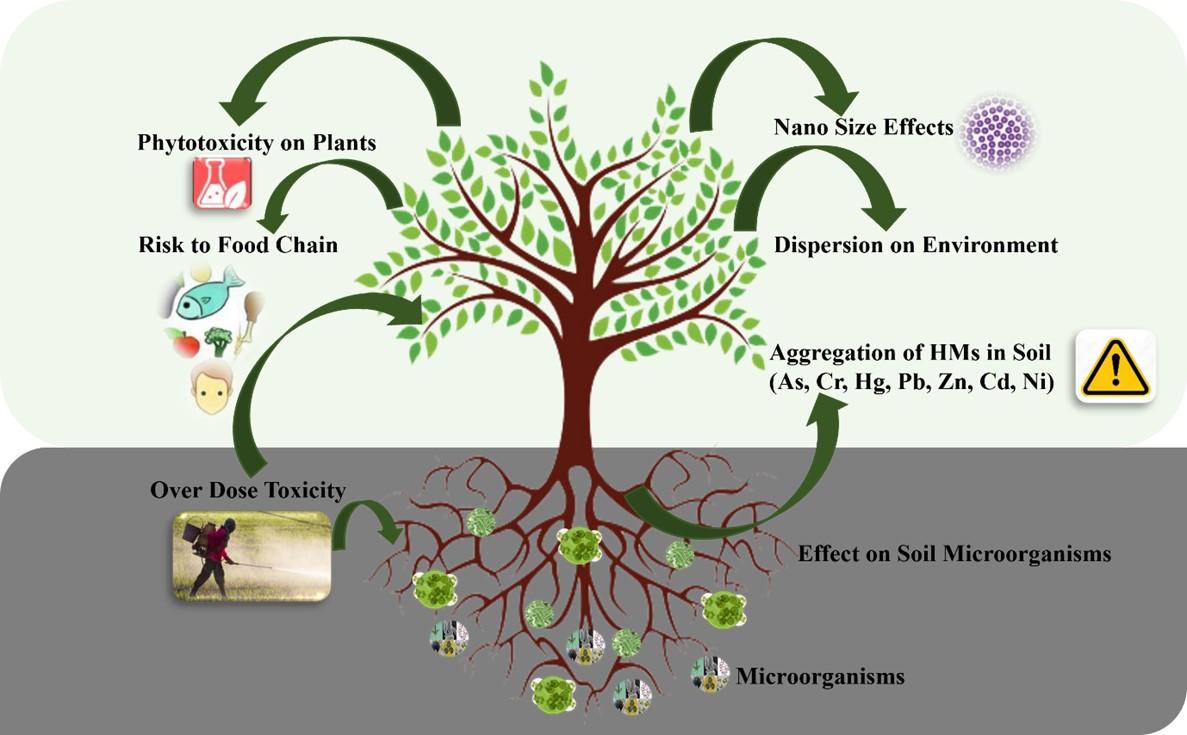
NANO-BIOREMEDIATION OF HEAVY METALS USING MICROORGANISMS
Nanoparticles-based bioremediation is an emerging and highly efficient approach for large-scale environmental cleanup, minimizing toxic repercussions. In the relentless march of technology, bioremediation has evolved into nano-bioremediation, employing nanoparticles and microbes to offer eco-friendly solutions for tackling hazardous environmental pollutants (Figure 3).
Microorganisms-based HMs removing or minimizing are more efficient approaches compared to traditional methods [50]. Metals like As, Cd, and Pb are highly toxic even at low concentrations [51]. Microbial bioremediation immobilizes these metals; for example, Morganella psychrotolerans produces silver nanoparticles for heavy metal removal [52], while iron oxide nanoparticles with polyvinyl pyrrolidone (PVP) and Halomonas sp. effectively remediate Pb and Cd [53]. Additionally, silica nanoparticles, Pseudomonas aeruginosa, and graphene oxide remove polycyclic aromatic hydrocarbons (PAHs) [54], and Halomonas immobilized with magnetic NPs degraded Pd metal [55]. Moreover, Bacteria synthesize diverse nanoparticles used for immobilization and mobilization of metals [56], with strains like Bacillus cereus (PMBL-3) and Lactobacillus macroides (PMBL-7) effectively remediating HMs such as Cd, Cr, Pd, and Cu [57]. Interestingly, Myconanotechnology employs fungi for bioremediation (Table 1), with various mushroom species effectively remediating soil contaminants [58, 59]. Fungi such as Fusarium solani are increasingly being used in the process of nanoparticle manufacturing, because of their resistance to heavy metals [60], while Trichoderma harzianum degrades pentachlorophenol, and Cryptococcus sp. displays resilience to HMs [61].
The chemical structure and molecular weight of microplastics (MPs), along with environmental conditions, influence microbial-driven degradation. This process involves biodeterioration, bio-fragmentation, biosynthesis, and mineralization [82]. Although the precise mechanism of Pseudomonas sp. in degrading MPs particles remains elusive, research suggests the involvement of chitinase degradation [83]. Microorganisms utilize metal-based nanoparticles (MNPs) as a carbon source for growth, aiding in the degradation of high molecular-weight plastics [84]. The microbial degradation of plastic fragments offers a green solution, but controlling changes in plastic pollution relies on various factors. Therefore, the utilization of effective microbes can be a suitable approach for eliminating MNPs [81, 85]. However, microbial degradation of MNPs is still in its infancy, as the characterization process is very slow, and incomplete mineralization is another limitation [86]. MNPs present in municipal solid waste (MSW) can harbor various microinorganic and organic pollutants, posing risks to the environment and human health as they enter the food chain [87]. Indigenous microbial communities within MSW and sewage sludge exhibit plastic degradation capabilities. Recent studies have shown that mesophilic Stenotrophomonas panacihumi can convert polypropylene (PP) into low and high-molecular-weight forms after 90 days [88]. The persistence of antibacterial nanoparticles beyond a threshold poses a threat to soil microbes, potentially inhibiting nitrogen-fixing microbes, leading to stunted plant growth and reduced production [89].
In the perspective of environmental bioremediation, leveraging nanotechnology alongside microbial assistance emerges as a highly efficient strategy, as evidenced by the effective removal of HMs and pollutants from soil and wastewater. While nano-bioremediation offers promising solutions, the persistence of antibacterial nanoparticles poses challenges to soil microbial communities and ecosystems. Despite advancements, microbial degradation of micro-nano plastics remains in its nascent stages, highlighting the need for further research to optimize biodegradation processes and minimize environmental risks.
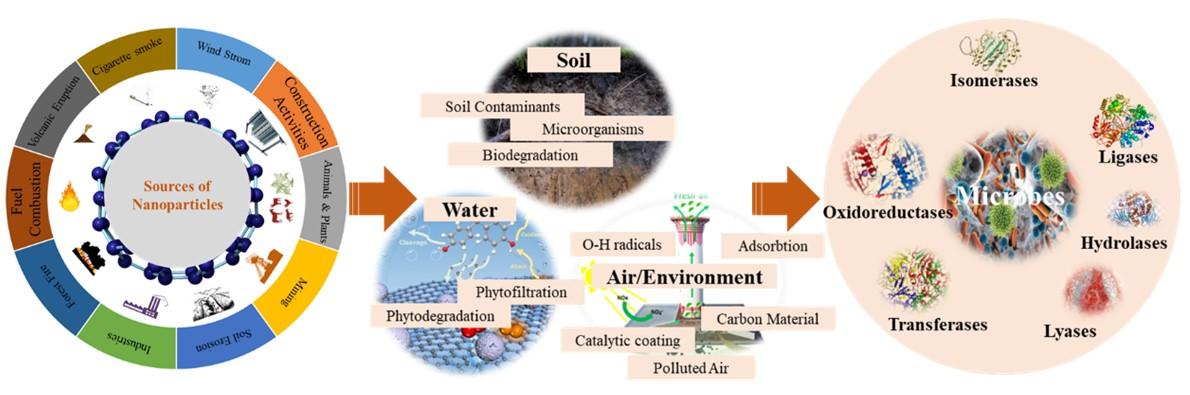
Table 1. Potential implication of microorganisms for bioremediation of HMs from plants, soils and environments.
LIMITATIONS AND FUTURE PROSPECTS
The application of microbes in remediation of HMs is known as nano-bioremediation and has drawn significant attention due to its efficient and environmentally friendly nature. However, several limitations have also been noticed during its widespread application. Saharan et al. [90] observed, the HMs toxicity in microbial communities affect the metabolic activity of these microorganisms and consequently their overall efficacy. In contrast, the environmental persistence of engineered NPs expands concerns about their potential ecotoxicological impacts, including bioaccumulation and non-target effects [91]. Laboratory findings often struggle to translate to field conditions due to variations in soil composition, pH, temperature, and competing ions, alongside unpredictable interactions among nanoparticles, plants, and microbes that are not fully understood [92, 93]. The characteristics of engineered nanoparticles can change due to agglomeration or dissolution, affecting their reactivity and potential toxicity [94]. Additionally, the long-term impacts of these nanoparticles, such as their accumulation in plant tissues or migration into water sources, remain poorly characterized. The scalability of nanoparticles is limited by expensive synthesis techniques and the high costs of large-scale field applications, especially in resource-constrained regions. Nanoparticles can introduce new environmental contaminants and potentially disrupt soil microbiomes, altering nutrient cycles and ecosystem balance due to high concentrations. The adoption of nanotechnology is complicated by inconsistent regulations across different regions and the absence of standardized protocols for assessing environmental safety and long-term impacts [95].
The environmentally friendly biodegradable nanoparticles will alleviate the worries about persistence and eco-toxicity effects [68]. Integration with omics technologies, particularly genomics and proteomics, will provide deeper insight into the interaction of microbes with nanoparticles, thereby facilitating the design of tailored bioremediation strategies for specific contaminants [96]. Real-time monitoring systems are integrated with nano-bioremediation, also including biosensors that enhance process efficiency while ensuring site-specific applicability [17]. Advancing the field of nano-bioremediation of heavy metals through plant-microorganism interactions involves several promising directions. Elucidating mechanisms at the molecular level using comprehensive omics approaches and real-time monitoring techniques, such as synchrotron-based spectroscopy, can support deep insights into processes involved in HM and nanoparticle transformations.
Developing eco-friendly and cost-effective nanomaterials through green synthesis methods and creating biodegradable nanoparticles can minimize environmental impact and production costs. Integrating nano-bioremediation with different remediation strategies, such as phytoremediation and chemical techniques, can enhance contaminant removal efficiency and manage complex pollution scenarios more effectively. Conducting field trials and validating long-term effectiveness under various conditions is crucial for assessing the feasibility and sustainability of nano-bioremediation methods. Additionally, the application of advanced models and tools, including AI and machine learning, can optimize remediation processes and customize approaches based on local environmental conditions. Harmonization and the establishment of standardized risk-benefit analyses are essential for gaining broader acceptance and ensuring the responsible implementation of nano-bioremediation technologies. These efforts will help refine nano-bioremediation strategies, making them more efficient, scalable, and environmentally safe. Therefore, the application of advanced nano-based tools and sustainable restoration of ecosystems from HMs-contaminated environments would be supportive for making smart and HMs-free green environments.
CONCLUSIONS
This study explores effective strategies for microorganism-assisted HMs remediation, the role of plant-microbe interactions for nano-bioremediation of HMs, and strategies for transforming toxic HMs into less toxic forms. This study updates the techniques like biomineralization, biostimulation, and mycoremediation, discusses how microbe-assisted phytoremediation is crucial for minimizing HMs contamination, and shows sustainable solutions for maintaining of HMs toxicity. This review also highlights the prospect of using nano-environmental biotechnology tools for mitigating HMs toxicity in plants, soils, and environments. This updated study with eco-friendly approaches to HMs removal from environments could be useful for minimizing HMs toxicity and converting to smart-green earth.
ACKNOWLEDGEMENTS
The authors would like to acknowledge the College of Agriculture, Food and Environmental Sciences, California Polytechnic State University, San Luis Obispo, USA for providing research facilities and assistance.
AUTHOR CONTRIBUTIONS
KD conceived the research plan, and supervised the study. KD, MAAM, and AS wrote the initial draft of the manuscript. KD, FMA, and SD edited the manuscript. All authors have approved the final version.
CONFLICTS OF INTEREST
There is no conflict of interest among the authors.
References
- [1]Ayangbenro AS, Babalola OO. A new strategy for heavy metal polluted environments: a review of microbial biosorbents. International Journal of Environmental Research and Public Health. 2017;14:94.
- [2]Tang H, Xiang G, et al. Microbial mediated remediation of heavy metals toxicity: mechanisms and future prospects. Frontiers in Plant Science. 2024;15:1420408.
- [3]Angon PB, Islam MS, et al. Sources, effects and present perspectives of heavy metals contamination: soil, plants and human food chain. Heliyon. 2024;10:e28357.
- [4]Verma JP, Jaiswal DK. Book review: Advances in biodegradation and bioremediation of industrial waste. Frontiers in Microbiology. 2016;6:1555.
- [5]Demnerova K, Mackova M, et al. Two approaches to biological decontamination of groundwater and soil polluted by aromatics characterization of microbial populations. International Microbiology. 2005;8:205–11.
- [6]Deepa CN, Suresha S. Biosorption of lead (II) from aqueous solution and industrial effluent by using leaves of Araucaria cookii: application of response surface methodology. Journal of Environmental Science, Toxicology and Food Technology. 2014;8(7):67–79.
- [7]Siddiquee S, Rovina K, et al. Heavy metal contaminants removal from wastewater using the potential filamentous fungi biomass: a review. Journal of Microbial & Biochemical Technology. 2015;7(6):384–95.
- [8]Igiri BE, Okoduwa SI, et al. Toxicity and bioremediation of heavy metals contaminated ecosystem from tannery wastewater: a review. Journal of Toxicology. 2018;1–16.
- [9]Zhang WJ, Jiang FB, et al. Global pesticide consumption and pollution: with China as a focus. PIAEES. 2011;1(2):125–44.
- [10]Ojuederie OB, Babalola OO. Microbial and plant-assisted bioremediation of heavy metal polluted environments: a review. International Journal of Environmental Research and Public Health. 2017;14:1504.
- [11]Nematian MA, Kazemeini F. Accumulation of Pb, Zn, C, and Fe in plants and hyperaccumulator choice in Galali iron mine area, Iran. International Journal of Agriculture and Crop Sciences. 2013;5:426.
- [12]Dixit R, Malaviya D, et al. Bioremediation of heavy metals from soil and aquatic environment: an overview of principles and criteria of fundamental processes. Sustainability. 2015;7:2189–212.
- [13]Strong PJ, Burgess JE. Treatment methods for wine-related and distillery wastewaters: a review. Bioremediation Journal. 2008;12:70–87.
- [14]Conesa HM, Evangelou MWH, et al. A critical view of current state of phytotechnologies to remediate soils: still a promising tool?. The Scientific World Journal. 2012;2012:173829.
- [15]Wang J, Chen C. Biosorbents for heavy metal removal and their future. Biotechnology Advances. 2009;27:195–226.
- [16]Jothimani P, Bhaskaran A. Assessing the azoreductase enzyme activity of bacterial cultures used for decolourisation. Journal of Ecotoxicology & Environmental Monitoring. 2003;13(3):179–83.
- [17]Ur Rahman S, Qin A, et al. Pb uptake, accumulation, and translocation in plants: plant physiological, biochemical, and molecular response: a review. Heliyon. 2024;10:e27724.
- [18]Rajkumar M, Ae N, et al. Potential of siderophore-producing bacteria for improving heavy metal phytoextraction. Trends in Biotechnology. 2010;28:142–9.
- [19]Ansari MI, Malik A. Biosorption of nickel and cadmium by metal-resistant bacterial isolates from agricultural soil irrigated with industrial wastewater. Bioresource Technology. 2007;98:3149–53.
- [20]Sutherland RA, Day JP, et al. Lead concentrations, isotope ratios, and source apportionment in road-deposited sediments, Honolulu, Oahu, Hawaii. Water, Air, and Soil Pollution. 2003;142:165–86.
- [21]Ozkutlu F, Turan M, et al. Assessment of heavy metal accumulation in the soils and hazelnut (Corylus avellana L.) from Black Sea coastal region of Turkey. Asian Journal of Chemistry. 2009;21:4371–88.
- [22]Hunter P. A toxic brew we cannot live without. EMBO Reports. 2008;9(1):15–8.
- [23]Zhao FJ, Ma JF, et al. Arsenic uptake and metabolism in plants. New Phytologist. 2009;181(4):777–94.
- [24]Verbruggen N, Hermans C, et al. Mechanisms to cope with arsenic or cadmium excess in plants. Current Opinion in Plant Biology. 2009;12:1–9.
- [25]Igwe JC, Nnorom IC, et al. Kinetics of radionuclides and heavy metals behavior in soils: implications for plant growth. African Journal of Biotechnology. 2005;4(B):1541–7.
- [26]Ortega-Larrocea MP, Siebe C, et al. Mycorrhizal inoculum potential of arbuscular mycorrhizal fungi in soils irrigated with wastewater for various lengths of time, as affected by heavy metals and available P. Applied Soil Ecology. 2007;37:129–38.
- [27]Zhuang W, Gao X. Acid-volatile sulfide and simultaneously extracted metals in surface sediments of the southwestern coastal Laizhou Bay, Bohai Sea: concentrations, spatial distributions and the indication of heavy metal pollution status. Marine Pollution Bulletin. 2013;76:128–38.
- [28]Pierzynski GM, Sims JT, et al. Soil and environmental quality. Boca Raton, United States of America: CRC Press; 2000.
- [29]Chakraborty S, Mukherjee A, et al. Biochemical characterization of a lead-tolerant strain of Aspergillus foetidus: an implication of bioremediation of lead from liquid media. International Biodeterioration and Biodegradation. 2013;84:134–42.
- [30]Kabata-Pendias A, Pendias H. Trace elements in soils and plants. London: CRC Press; 2001.
- [31]Perpetuo EA, Souza CB, et al. Engineering bacteria for bioremediation. In: Carpi A, editor. Progress in molecular and environmental bioengineering: from analysis and modeling to technology applications. Rijeka: InTech; 2011. p. 605–32.
- [32]López-Vargas ER, Ortega-Ortíz H, et al. Foliar application of copper nanoparticles increases the fruit quality and the content of bioactive compounds in tomatoes. Applied Sciences. 2018;8(7):1020.
- [33]Hamzah Saleem M, Usman K, et al. Functions and strategies for enhancing zinc availability in plants for sustainable agriculture. Frontiers in Plant Science. 2022;13:1033092.
- [34]Ventrella A, Catucci L, et al. Interactions between heavy metals and photosynthetic materials studied by optical techniques. Bioelectrochemistry. 2009;77:19–25.
- [35]Yan A, Wang Y, et al. Phytoremediation: a promising approach for revegetation of heavy metal-polluted land. Frontiers in Plant Science. 2020;11:359.
- [36]Hassan MU, Chattha MU, et al. Nickel toxicity in plants: reasons, toxic effects, tolerance mechanisms, and remediation possibilities—a review. Environmental Science and Pollution Research. 2019;26:12673–88.
- [37]Angon PB, Islam MS, Kc S, et al. Sources, effects and present perspectives of heavy metals contamination: Soil, plants and human food chain. Heliyon. 2024;10(7):e28357.
- [38]Khalid S, Shahid M, et al. A comparison of technologies for remediation of heavy metal contaminated soils. Journal of Geochemical Exploration. 2017;182:247–68.
- [39]Liu CJ, Deng SG, et al. Applications of bioremediation and phytoremediation in contaminated soils and waters: CREST publications during 2018–2022. Critical Reviews in Environmental Science and Technology. 2023;53:723–32.
- [40]El-Sappah AH, Zhu Y, et al. Plants’ molecular behavior to heavy metals: from criticality to toxicity. Frontiers in Plant Science. 2024;15:1423625.
- [41]Hama Aziz KH, Mustafa FS, et al. Heavy metal pollution in the aquatic environment: efficient and low-cost removal approaches to eliminate their toxicity: a review. RSC Advances. 2023;13:17595–17610.
- [42]Abd Elnabi MK, Elkaliny NE, et al. Toxicity of heavy metals and recent advances in their removal: a review. Toxics. 2023;11:580.
- [43]Podar D, Maathuis FJM. The role of roots and rhizosphere in providing tolerance to toxic metals and metalloids. Plant, Cell & Environment. 2022;45:719–36.
- [44]Khan F, Siddique AB, et al. Phosphorus plays key roles in regulating plants’ physiological responses to abiotic stresses. Plants. 2023;12(15):2861.
- [45]Sun S-C, Chen JX, et al. Molecular mechanisms of heavy metals resistance of Stenotrophomonas rhizophila JC1 by whole genome sequencing. Archives of Microbiology. 2021;203:2699–709.
- [46]Rajput VD, Minkina T, et al. Nanotechnology in the restoration of polluted soil. Nanomaterials. 2022;12(5):769.
- [47]Sharma B, Sarkar A, et al. Agricultural utilization of biosolids: a review on potential effects on soil and plant growth. Waste Management. 2017;64:117–32.
- [48]Alengebawy A, Abdelkhalek ST, et al. Heavy metals and pesticides toxicity in agricultural soil and plants: ecological risks and human health implications. Toxics. 2021;9:42.
- [49]Kladsomboon S, Jaiyen C, et al. Heavy metals contamination in soil, surface water, crops, and resident blood in Uthai District, Phra Nakhon Si Ayutthaya, Thailand. Environmental Geochemistry and Health. 2019;42:545–61.
- [50]Singh Y, Saxena MK. Insights into the recent advances in nano-bioremediation of pesticides from the contaminated soil. Frontiers in Microbiology. 2022;13:982611.
- [51]Bist P, Choudhary S. Impact of heavy metal toxicity on the gut microbiota and its relationship with metabolites and future probiotics strategy: a review. Biological Trace Element Research. 2022;200(12):5328–50.
- [52]Enez A, Hudek L, et al. Reduction in trace element-mediated oxidative stress towards cropped plants via beneficial microbes in irrigated cropping systems: a review. Applied Sciences. 2018;8(10):1953.
- [53]Alabresm A, Chen YP, et al. A novel method for the synergistic remediation of oil-water mixtures using nanoparticles and oil-degrading bacteria. Science of the Total Environment. 2018;630:1292–1297.
- [54]Rajput VD, Kumari S, Minkina T, Sushkova S, Mandzhieva S. Nano-enhanced microbial remediation of PAHs contaminated soil. Air, Soil and Water Research. 2023;16:1–7.
- [55]Cao X, Alabresm A, et al. Improved metal remediation using a combined bacterial and nanoscience approach. Science of the Total Environment. 2020;704:135378.
- [56]Pandit C, Roy A, et al. Biological agents for synthesis of nanoparticles and their applications. Journal of King Saud University – Science. 2022;34(3):101869.
- [57]Mane PC, Bhosle AB. Bioremoval of some metals by living algae Spirogyra sp. and Spirullina sp. from aqueous solution. International Journal of Environmental Research. 2012;6(2):571–6.
- [58]Bala S, Garg D, et al. Recent strategies for bioremediation of emerging pollutants: a review for a green and sustainable environment. Toxics. 2022;10:484.
- [59]Hu Y, Mortimer PE, et al. Mushroom cultivation for soil amendment and bioremediation. Circular Agricultural Systems. 2021;1(1):1–14.
- [60]Rasha FM. Intracellular siderophore detection in an Egyptian cobalt-treated isolate using SEM-EDX with reference to its tolerance. Polish Journal of Microbiology. 2017;66(2):235–43.
- [61]Singh MP, Vishwakarma SK, et al. Bioremediation of direct blue 14 and extracellular ligninolytic enzyme production by white rot fungi: Pleurotus spp. BioMed Research International. 2013;2013:180156.
- [62]Armendariz AL, Talano MA, et al. Impact of double inoculation with Bradyrhizobium japonicum E109 and Azospirillum brasilense Az39 on soybean plants grown under arsenic stress. Plant Physiology and Biochemistry. 2019; 138:26–35.
- [63]Bruno LB, Anbuganesan V, et al. Enhanced phytoextraction of multi-metal contaminated soils under increased atmospheric temperature by bioaugmentation with plant growth-promoting Bacillus cereus. Journal of Environmental Management. 2021; 289:112553.
- [64]Kumar A, Voropaeva O, et al. Bioaugmentation with copper-tolerant endophyte Pseudomonas lurida strain EOO26 for improved plant growth and copper phytoremediation by Helianthus annuus. Chemosphere. 2021; 266:128983.
- [65]Ahluwalia SS, Goyal D. Microbial and plant-derived biomass for removal of heavy metals from wastewater. Bioresource Technology. 2007;98(12):2243–2257.
- [66]Jeyasundar PGSA, Ali A, et al. Green remediation of toxic metals contaminated mining soil using bacterial consortium and Brassica juncea. Environmental Pollution. 2021; 277:116789.
- [67]Jampasri K, Pokethitiyook P, et al. Bacteria-assisted phytoremediation of fuel oil and lead co-contaminated soil in the salt-stressed condition by Chromolaena odorata and Micrococcus luteus. International Journal of Phytoremediation. 2020; 22:322–33.
- [68]Yang X, Qin J, et al. Upland rice intercropping with Solanum nigrum inoculated with arbuscular mycorrhizal fungi reduces grain cadmium while promoting phytoremediation of cadmium-contaminated soil. Journal of Hazardous Materials. 2021; 406:124325.
- [69]Irshad S, Xie Z, et al. Indigenous strain Bacillus XZM-assisted phytoremediation and detoxification of arsenic in Vallisneria denseserrulata. Journal of Hazardous Materials. 2020; 381:120903.
- [70]Chaturvedi R, Favas PJ, et al. Harnessing Pisum sativum-Glomus mosseae symbiosis for phytoremediation of soil contaminated with lead, cadmium, and arsenic. International Journal of Phytoremediation. 2021; 23:279–90.
- [71]Machado MD, Soares EV, et al. Removal of heavy metals using a brewer’s yeast strain of Saccharomyces cerevisiae: chemical speciation as a tool in the prediction and improving of treatment efficiency of real electroplating effluents. Journal of Hazardous Materials. 2010;180(1–3):347–53.
- [72]Kullu B, Patra DK, et al. AM fungi-mediated bioaccumulation of hexavalent chromium in Brachiaria mutica: A mycorrhizal phytoremediation approach. Chemosphere. 2020; 258:127337.
- [73]Lee YC, Chang SP. The biosorption of heavy metals from aqueous solution by Spirogyra and Cladophora filamentous macroalgae. Bioresource Technology. 2011;102(9):5297–304.
- [74]Manyangadze M, Chikuruwo NHM, et al. Enhancing adsorption capacity of nano-adsorbents via surface modification: a review. South African Journal of Chemical Engineering. 2020;31(1):25–32.
- [75]Tripathi P, Khare P, et al. Bioremediation of arsenic by soil methylating fungi: role of Humicola sp. strain 2WS1 in amelioration of arsenic phytotoxicity in Bacopa monnieri L. Science of the Total Environment. 2020; 716:136758.
- [76]Saunders RJ, Paul NA, et al. Sustainable sources of biomass for bioremediation of heavy metals in wastewater derived from coal-fired power generation. PLoS ONE. 2012;7(5):e36470.
- [77]Cantamessa S, Massa N, et al. Phytoremediation of a highly arsenic-polluted site, using Pteris vittata L. and arbuscular mycorrhizal fungi. Plant Theory. 2020; 9:1211.
- [78]Saeed-ur-Rahman MK, Kayani SI, et al. The ameliorative effects of exogenous inoculation of Piriformospora indica on molecular, biochemical, and physiological parameters of Artemisia annua L. under arsenic stress condition. Ecotoxicology and Environmental Safety. 2020; 206:111202.
- [79]You Y, Wang L, et al. Effects of arbuscular mycorrhizal fungi on the growth and toxic element uptake of Phragmites australis under zinc/cadmium stress. Ecotoxicology and Environmental Safety. 2021; 213:112023.
- [80]Złoch M, Kowalkowski T, et al. Modeling of phytoextraction efficiency of microbially stimulated Salix dasyclados L. in soils with different speciation of heavy metals. International Journal of Phytoremediation. 2017; 19:1150–64.
- [81]Fan M, Xiao X, et al. Enhanced phytoremediation of Robinia pseudoacacia in heavy metal-contaminated soils with rhizobia and the associated bacterial community structure and function. Chemosphere. 2018; 197:729–40.
- [82]Tiwari N, Santhiya D, et al. Microbial remediation of micro-nano plastics: current knowledge and future trends. Environmental Pollution. 2020;265:115044.
- [83]Rogers KL, Carreres-Calabuig JA, et al. Micro-by-micro interactions: how microorganisms influence the fate of marine microplastics. Limnology and Oceanography. 2020;5:18–36.
- [84]Othman AR, Hasan HA, et al. Microbial degradation of microplastics by enzymatic processes: a review. Environmental Chemistry Letters. 2021;1–17.
- [85]Knott BC, Erickson MD, et al. Characterization and engineering of a two-enzyme system for plastics depolymerization. Proceedings of the National Academy of Sciences. 2020;117(41):25476–85.
- [86]Anastopoulos I, Pashalidis I. Single-use surgical face masks, as a potential source of microplastics: do they act as pollutant carriers?. Journal of Molecular Liquids. 2021;326:115247.
- [87]Golwala H, Zhang X, et al. Solid waste: an overlooked source of microplastics to the environment. Science of the Total Environment. 2021;144581.
- [88]Ru J, Huo Y, et al. Microbial degradation and valorization of plastic wastes. Frontiers in Microbiology. 2020;11:442.
- [89]Beddow J, Stolpe B, et al. Effects of engineered silver nanoparticles on the growth and activity of ecologically important microbes. Environmental Microbiology Reports. 2014;6(5):448–58.
- [90]Saharan BS, Chaudhary T, Mandal BS, Kumar D, Kumar R, Sadh PK, Duhan JS. Microbe-plant interactions targeting metal stress: new dimensions for bioremediation applications. Journal of Xenobiotics. 2023;13(2):252-269.
- [91]Manganyi MC, Dikobe TB, Maseme MR. Exploring the potential of endophytic microorganisms and nanoparticles for enhanced water remediation. Molecules. 2024;29(12):2858.
- [92]Ahmed T, Noman M, et al. Dynamic interplay between nano-enabled agrochemicals and the plant-associated microbiome. Trends in Plant Science. 2023;28(11):1310–25.
- [93]Masud MAA, Shin WS. Advanced carbo-catalytic degradation of antibiotics using conductive polymer-seaweed biochar composite: exploring N/S functionalization and non-radical dynamics. Journal of Hazardous Materials. 2024;478:135449.
- [94]Wang D, Pan Q, et al. Effects of mixtures of engineered nanoparticles and cocontaminants on anaerobic digestion. Environmental Science & Technology. 2024;58(6):2598–614.
- [95]Shemawar, Mahmood A, Hussain S, Mahmood F, Iqbal M, Shahid M, Ibrahim M, Ali MA, Shahzad T. Toxicity of biogenic zinc oxide nanoparticles to soil organic matter cycling and their interaction with rice-straw derived biochar. Scientific Reports. 2021;11(1):8429.
- [96]Singh Y, Saxena MK. Insights into the recent advances in nano-bioremediation of pesticides from the contaminated soil. Frontiers in Microbiology. 2022;13:982611.