Utilization of endophytic bacteria for liquid biofertilizer production with a newly designed prototype fermentor for plant improvement
Abstract
Endophytic bacteria are a promising source of cost-effective and eco-friendly approaches that are highly desirable for sustainable agriculture. The study successfully developed a novel prototype fermentor and demonstrated an impactful utilization of endophytic bacteria for large-scale production of biofertilizers. The fermentor was designed to integrate i) a temperature control unit, ii) a heating unit, a heated water circulation unit, and iii) a microbial growth unit, ensuring precise control of temperature and agitation, providing a conducive environment containing an affordable medium for the proliferation of single endophytic bacterium, or consortium of endophytic bacteria. The capacities of the fermentor revealed that the temperature of the growth medium could be elevated from 29°C to 37°C within 50 minutes at varying rotation speeds of 60 rpm, 75 rpm, or 90 rpm, securing uniform temperature distribution for optimum growth of the consortia. Each consortium's average growth rate was recorded after 48 hours. Furthermore, the system efficiently returned to the initial temperature of 29°C from 37°C within 4.5 hours and maintained a temperature around 35°C to 37°C during a brief period of over ~70 minutes of power outage. The amplified culture of consortium A. comprised of growth-promoting endophytic bacteria (Klebsiella sp. HSTU-Bk11, Acinetobacter sp. HSTU-Abk29, Citrobacter sp. HSTU-ABk30, and Enterobacter cloacae HSTU-ABk39) significantly improved the morphological traits of rice plants (root, shoot, tillers number) along with the crop yield in the fields. This study presents a novel fermentor, facilitating the large-scale production of plant growth-promoting endophytic bacteria, and presenting new insights for enhancing sustainable organic agricultural research and industrial applications.
INTRODUCTION
The world's population is projected to reach 9.7 billion by the year 2050 and 11 billion by the year 2100 [1] with an annual growth rate of 0.88% [2], making access to healthy nutrition unreachable, raising a critical concern. The global demand for food is predicted to increase by 35% to 56% from 2010 to 2050, while the proportion of people at risk of hunger is expected to shift from a negative 91% to a positive 8% during the same period [3, 4]. To augment the agricultural output to meet the increased demand along with the scarcity of available lands, farmers faced critical choices, including the use of chemical fertilizers/pesticides more and more to increase crop production. The excessive applications of chemical fertilizers over time degrade soil properties and lower soil fertility [5]; increase water pollution, and alter soil pH acidity, causing a decrease in the microbial population in the rhizosphere and a rise in the vulnerability of the crops to insects [6]. Furthermore, rice production in Bangladesh heavily depends on the use of fertilizers with an average application rate of over 286 kg/hectare. The country imports over 1.2 million tons of chemical fertilizers every year, meeting the needs of 31%, 57%, and 95% of nitrogen, phosphate, and potash, respectively [7], and impacting the foreign reserves. On the other hand, organic fertilizers enhance the soil's fertility, physical and chemical properties, ability to retain water, fungal-to-bacterial ratio, and soil enzyme activity, hence crop yields [8]. The efficient use of plant growth-promoting rhizobacteria (PGPR) in agriculture has been demonstrated to replace the application of chemical fertilizers and subsequently help the development of eco-friendly crop production [9, 10]. Furthermore, plant growth, root development patterns, competitiveness in obtaining nutrition from the soil, and defense against biotic and abiotic stresses can be improved by plant growth-promoting rhizobacteria (PGPR) application [10-13]. Many rhizospheric bacteria, including Azospirillum, Bacillus, Pseudomonas, and Enterobacter have been employed to increase crop production under various stress conditions [14-16]. Rhizospheric bacteria, such as Pseudomonas sp., Azospirillum sp., Pantoea sp., and Agrobacterium sp. enhanced nutrient absorption under mild temperatures by several crops, including maize, wheat, and legumes, and subsequent yields [17]. These PGPRs not only have the capacity to colonize plant roots, benefiting their hosts by regulating phytohormone synthesis, enhancing the availability of soil minerals, and enhancing disease resistance, but also can act as phytostimulators, changing the metabolism of phytohormones by increasing the synthesis of auxin, cytokinins, abscisic acid, gibberellins, and by reducing ethylene [18-20]. In addition to reducing the use of chemical fertilizers, PGPR has been reported to enhance crop production by effectively mitigating biotic and abiotic factors and abiotic stresses [21-23]. Through competition for nutrients, antagonistic relationships, and the induction of systemic resistance, PGPR also indirectly serves as biopesticides or biocontrol agents, enhancing resistance to phytopathogens [18, 19, 23].
Under ideal conditions, a bacterium growing on a homogenous rich culture medium can multiply in as little as twenty minutes [24]. On the other hand, it has been proposed that in some deep terrestrial habitats, cell division might happen as slowly as once per 100 years [25] and has been attributed to the numerous variables, including nutrient-poor subsurface ecosystems responsible for the delayed development of cells. Consequently, cells are more likely to remain isolated, unable to exchange nutrients or activate defense systems, and grow more slowly [25]. Thus, a system enabling regulated changes with desired precision under various circumstances is essential for the large-scale production of bacteria to be used as bio-fertilizers. Furthermore, the system a fermentor, provides a safe and repeatable technological resource required to conduct carefully thought-out studies aimed at understanding the biological, chemical, and/or physical effects on the uniform growth of the bacterial consortium. An effective fermentor offers advantages in terms of minimal risk of contamination, simplicity of use, and scalability for eventual applications in the fields. To date, due to the design complexity of the fermentor, the output objectives and expectations in the process of developing the system have only been met partially [26]. To promote optimal bacterial growth, a regulated microenvironment with the ability of precise mechanical stimulation is essential. Through effective mixing, ideal mass, and heat transmission, along with a regulated growth environment, such as temperature, the fermentor is intended to do its purpose efficiently, providing an ideal condition for enhancing bacterial culture. To prevent inappropriate mixing, resulting in a heterogeneous environment and inhibition of growth [27], the fermentor should be simple to scale up the production of biofertilizers. However, scaling up biofertilizer production presents multiple challenges, such as achieving high cell concentrations, ensuring product safety by removing contaminants, and lowering production costs [28]. On the other hand, the production of biofertilizers (consortia of endophytic bacteria) can be costly due to the expensive media along with the available fermentors with limited capacity to culture the bacterial consortium. Traditional media used for large-scale cultures of bacterial biofertilizer is typically made from expensive ingredients, such as peptone and yeast extract. Hence, we have developed a new inexpensive growth medium and designed a new fermentor, demonstrable for large-scale bacterial cultures, meeting the needs of next-generation biofertilizers for sustainable, cost-effective, and environmentally friendly organic agriculture. The medium is made from readily available cheap ingredients, such as poultry feed, maize flour, and wheat flour.
We have previously reported that the yield of rice grains was increased by 30-35% with a foliar spray of endophytic consortia [29, 30] and the production of tomatoes was increased by 2.5 folds with a foliar spray of Enterobacter sp. strain HSTU-Sh6 [30]. However, the production scale of the biofertilizers to cover the large area of farmlands was limited due to the use of the flask culture method. At present, the large-scale production of biofertilizers is greatly lacking in Bangladesh and worldwide due to a lack of needed technologies. This demands to development of a new cost-effective commercial fermentor to scale up the production of liquid-based endophytic bacterial consortia as biofertilizers. To this effect, the newly invented prototype fermentor reported here has the potential to revolutionize the production of biofertilizers, making it more affordable and accessible to farmers worldwide. Our group built the new fermentor in the consortium at a low cost with individual temperature, and agitation, and is suitable for use in undeveloped areas where outage of electricity is frequent.
MATERIALS AND METHODS
Design and fabrication of a new fermentor
A fermentor was newly designed comprised of multiple components, and is capable of producing repeatable outcomes with precision. These components were made from different materials with various shapes and sizes, depending on the specific requirements of the design. Different parts were assembled by cutting, drilling, and welding, which was required based on the availability of the resources [27]. For our prototype, we chose a 2 mm sheet of stainless steel to construct the fermentor because of its lasting resistance to rust, corrosion, and discoloration, maintaining a hygiene and sanitary milieu essential for bacterial growth and culture [31]. In addition, the flat, non-porous surface of stainless steel is naturally resistant to bacterial adhesion, making it simpler to manage and monitor bacterial proliferation without interference from the material [32]. Overall, these features of stainless steel are especially important in maintaining integrity, producing reliable results, and reducing the risk of contamination. The fermentor (Figure 1) was introduced includes a power source, an inner chamber defined as a sample enclosure, and an outer chamber. An access is included through which a test sample is placed within the inner chamber. The outer chamber wraps around one or more walls of the inner chamber. A temperature sensor (RTD PT100) was included in the fermentor that monitors the temperature of the inner chamber. The feature of the fermentor consisted of a heater and a pump connected to the outer chamber, circulating working fluid through the heater and the outer chamber and maintaining the temperatures of the inner chamber. In addition, a rotor blade was located within the inner chamber and connected to an electric motor; and a controller was configured to regulate the temperature within the inner chamber, the temperature of the working fluid, and the rotation speed of the electric motor.
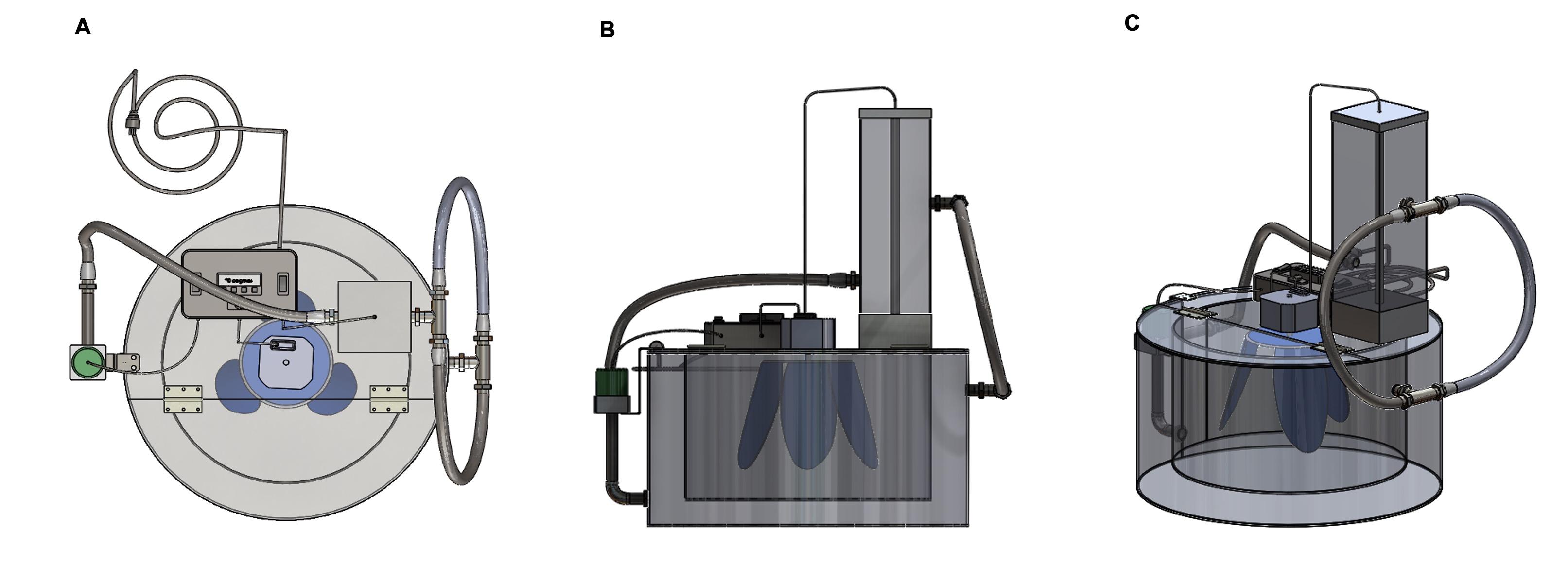
Description of the invention
The invention relates to the highly effective reliability of outputs with precision and a cost-efficient growth system with a significant decrease in production costs for biofertilizers (a consortium of bacterial cultures). The system was designed to produce approximately 25 liters of biofertilizer per production cycle. The heating system was embedded, and the temperature can be regulated, ranging from ambient temperature to 42°C. Furthermore, the speed of the rotor blade was adjusted through the RPM controller. The whole system operated from a 210-230V, 50-60Hz power source. This made the system user-friendly so that farmers could easily operate the setup under any load conditions in the rural areas of Bangladesh, where a limited supply of electricity is prevalent. Certain terminology was used to describe the instrument as depicted in Figure 2 (A-E) for convenience only, and the numbering refers to the component of the instrument. In drawing, the terms ‘right,’ ‘left,’ ‘top,’ and ‘bottom' define the directional references. In addition, the words ‘a’ and ‘one' are noted as one or more of the items included unless specifically stated otherwise.
The invention of a fermentor with a rotary motion and Heating system for the production of biofertilizers depicted in Figure 2 (A-E) contains (A) an outer chamber 1 made of aluminum, supporting the structure of the setup; (B) an inner bacterial growth chamber 3 made of aluminum – the working, chamber of the setup. The two chambers are welded together using an aluminum seal 2, keeping an equally distributed gap between the walls of the chambers. The temperature of the inner chamber is controlled by working fluid that flows between the gaps of the inner and outer chambers. Water is considered a working fluid that transfers heat from chamber 1 to chamber 3. The water was heated in heating chamber 7 (covered by a hood 6) and placed on fixed plate 5 of working chamber 3. The hot water was circulated between the two chambers by using a water pump 4. A set of pipes is connected between the water circulation area between chambers 1 and 3 through a 12-volt DC water pump 14 to the heating chamber 7. The water pump 14 was attached to the outer chamber 1 through a pump holder 15. The heating chamber 7 was fixed with the top fixed plate 5 through a chamber holder 8. A water heater 9 was placed inside the water heating chamber 7 and was regulated by a control panel 11 (AC 200-watt resistance heater) through a connecting wire 24. A rotor blade 13 was installed inside the growth chamber 3 and is connected to a 220V AC motor 12 operated by an AC power supply. This rotor blade 13 provides the rotary motion to the bacterial culture inside chamber 3. The rotor blade 13 is made of plastic so that it would not harm the bacteria growing inside chamber 3. A temperature sensor (RTD PT100) was installed inside chamber 3, recording the temperature of the culture medium, which needs to be controlled. The system was monitored by control unit 11 which harvests the signal from the temperature sensor installed inside chamber 3. The inner chamber 3 can be closed by a foldable hood 4 and the growth medium along with the bacterial inoculum can be inserted in the chamber by folding the hood. This foldable hood 4 was attached to the fixed hood 5 through a set of hinges 25. A set of fittings like flexible pipe 10, the pump inlet pipe 17, connecting pipe 18, tank fitting pipe 22, and different sets of joints 19, 20, and 21 were used for water circulation throughout the system. For power supply one main power cord 16 was connected to the control unit 11 which supplies power to the heater 9 and water pump 14 sequentially through connecting wires 23 and 24. Rotor blade 13 was powered through the main power cord 16, while a current regulator was added in the connection of the wire to the rotor blade 13 to control the speed of the rotor blade. All sets of connections were placed on the fixed plate 5. The temperature control unit of the system includes a temperature controller which was added to control unit 11, which has an external SSR (Solid State Relay) to realize a high heating power rate with optional PID (proportional–integral–derivative) control. Also, this device has an LCD (Liquid Crystal Display) with control switches for convenient operation. The controller has 250V AC 7.5A load for output and 20mA/10V DC Max load for SSR drive output. An NTC (Negative Temperature Coefficients), 5K/3470 sensor for temperature measurement was set. The operation range for temperature is 0°C -100°C with ± 1°C accuracy.
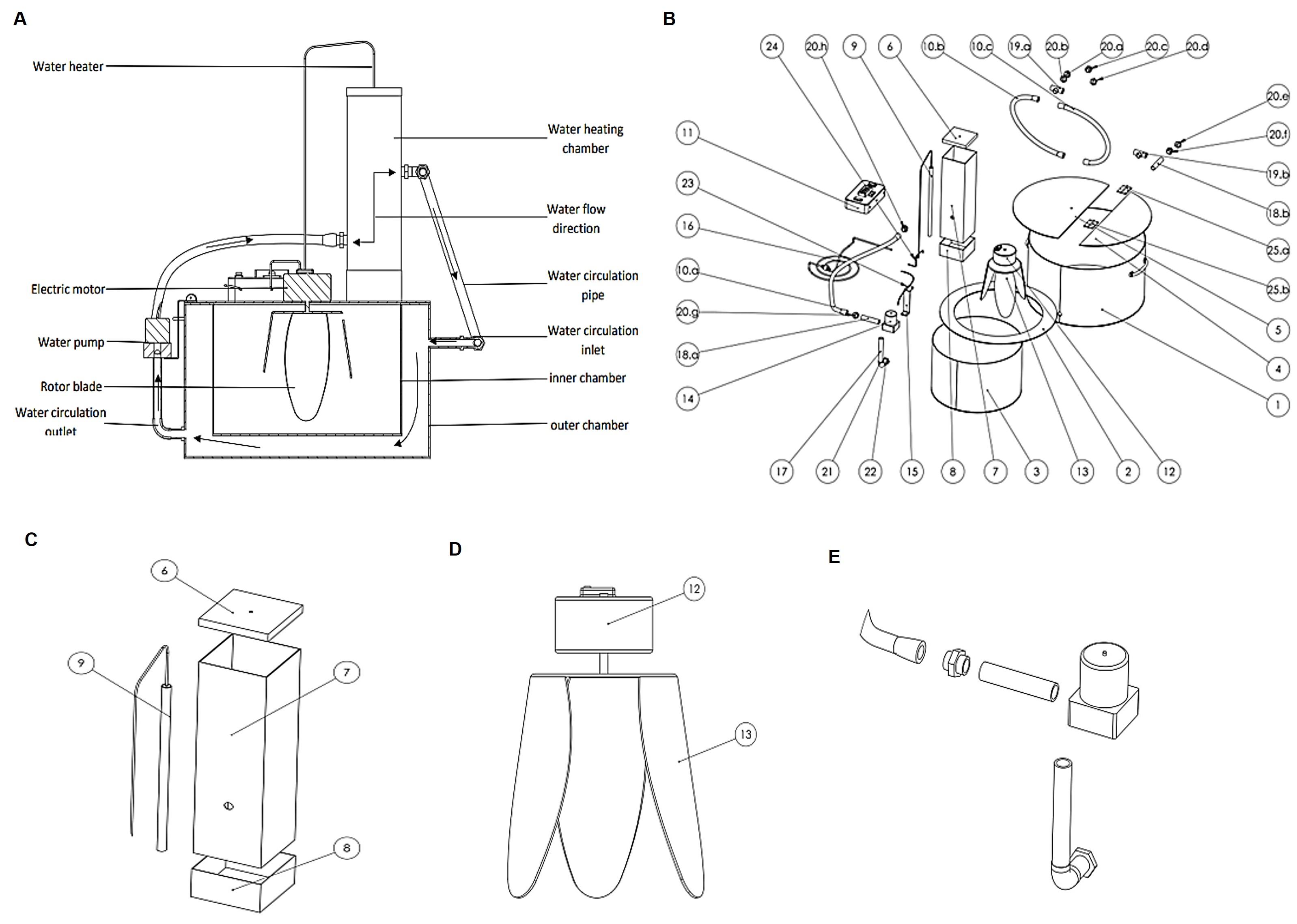
Working principle
To keep the temperature consistent, the system connects a temperature controller to the water heater and a sensor probe, taking the temperature reading directly from the bacterial growth chamber 3 (Figure 2) while the water heater warms the water inside the heating chamber. The heating chamber, measuring 5 inches in length, 5 inches in width, and 12 inches in height, is filled with water. The water was heated by an induction water heater rated at 1500 watts. The heated water leaves the chamber through the outlet and enters the water jacket via a flexible pipe, causing the uniform heating of the bacterial culture in the chamber 3 (2). This heated water then circulates and transfers heat to the bacterial growth chamber until the desired temperature is reached. In addition, a propeller continuously rotates inside the chamber, preventing the bacterial bodies from conjugating simultaneously, ensuring the bacterial mixture is evenly heated, and allowing the bacteria to grow at a consistent rate.
Validation of temperature stability of the growth chamber
Three primary modes of energy transfer involve the movement of heat between two systems with different temperatures. The first mode was heating conduction, which refers to the transfer of heat through atomic vibration, typically in solid objects. The second mode was heating convection, which involves the transfer of heat through mass flow between systems and was a significant factor in heat transfer in liquid systems. The third mode was heat radiation, which involves the emission of electromagnetic radiation from any object with a temperature higher than absolute zero (-273.15℃/0 K) [34, 35]. It was important to select the material used to build the chamber based on its thermal conductivity, calculated by determining the amount of heat that passes through a unit thickness of the material per unit area, given a specific temperature difference as previously reported [36].
We started the measurement of temperatures with our known temperature controller, but to check their accuracy, we used the Nicety DT1312 digital 2 input K-type thermometer. We recorded temperature values ranging from 28°C to 42°C, methodically regulating the agitator fan speeds in the chamber to 60, 75, and 90 rpm. Each temperature measurement was taken precisely to the closest 0.5°C, using the accuracy of the thermometer and a timer to track the time. We methodically recorded the temperature transitioning from 42°C to 28°C and noted every 0.5 increment change. This procedure allowed us to create a complete set of data, and to record with precision the intricate interplay between the temperature and fan speed within the chamber at any given time, achieving the desirable bacterial growth.
Nutrient substrates and bacterial consortia
The bacterial growth chamber was carefully assembled with the immobilizing structures and subjected to a thorough sterilization process to ensure the purity of the culture. The ingredients were mixed with 25 liters of water in a chamber and maintained at 37°C with agitation of 90 rpm, ensuring proper mixing and avoiding sedimentation. We have developed a new, affordable growth medium for large-scale cultures of next-generation bacterial biofertilizers. The medium consists of the ingredients with the amount as shown in Table 1.
The following four endophytic bacterial consortia [29] previously reported for rice plant growth were tested for their growth suitability in the newly assembled bacterial growth chamber for 72 h at 37ºC: Consortium A: Klebsiella sp. HSTU-Bk11 (MK656947.1), Acinetobacter sp. HSTU-Abk29 (MK695711.1), Citrobacter sp. HSTU-Abk30 (MK695712.1), and Enterobacter cloacae HSTU-Abk39 (MN795715); Consortium B: Enterobacter cloacae HSTU ABk37 (MN795712), Enterobacter ludwigii HSTU-Abk40 (MN795716), Acinetobacter baumannii HSTU-ABK42 (MN795722), Klebsiella sp. HSTU-ABk31 (MK695713.1), Acinetobacter sp. HSTU-Bk12 (MK656948.1); Consortium C: Pseudomonas sp. HSTU-Bk13 (MK656949.1), Citrobacter sp. HSTU-Bk14 (MK656950.1), Acinetobacter sp. HSTU-Bk15 (MK656951.1), Acinetobacter sp. HSTU-ABk32 (MN559060.1), and Burkholderia sp. HSTU-ABK33 (MK695714.1); and Consortium D: Acinetobacter sp. HSTU-ABk34 (MK695715.1), Enterobacter sp. HSTU-ABk36 (MN795711), Enterobacter sp. HSTU-ABk38 (MN795713), and Serratia marcescens HSTU-ABk41 (795720). These strains were chosen for their compatibility in the consortium as previously reported [29]. Each isolate was grown in nutrient broth for 24 h (106 CFU/mL for rice plants). We have used corn powder with commercial tryptic soya broth (TSB) and yeast extract (YE) powder in varied amounts (5-10 gm/25 liters) as reported previously [29]. In addition, the growth of the tomato plant growth-promoting bacteria, Enterobacter sp. strain HSTU-ASh6 [30] was conducted as described for the consortia of the endophytic bacteria for 72 h at 37ºC.
Table 1. Culture medium ingredients.
Application of biofertilizer in plants
We have applied twice the amplified bacterial consortia-A to rice plants (var. BRRI dhan28) in fields where nitrogen fertilizer inputs were reduced by 60–80% of the recommended doses in order to demonstrate the effectiveness of the biogrowth chamber on the production of biofertilizer. The biofertilizer's ability to reduce nitrogen fertilizer was measured, as were the morphological growth parameters (plant heights, root length, shoot length, leaf breadth, and chlorophyll contents).
RESULTS
Thermal stability of growth chamber
Figure 3 depicts the relationship between time and temperature for reaching the set value temperature (37°C) at three different agitation speeds of 60 rpm, or 75 rpm, or 90 rpm. The fastest gaining of preset temperature (37°C) was recorded to be at 90 rpm, followed by 75 rpm and then 60 rpm. A consistent trend of less than an hour to reach 37°C was recorded, indicating high efficiency and consistency of the system. The linear progression of time taken, and temperature rise implied a controlled and predictable heating process, demonstrating the stability and reliability of the system. This is critical for precise conditions required for the bacterial culture (production of bio-fertilizer), where temperature control is essential for optimal growth [37].
To examine the stability of temperature at the time of power disruption, a thorough examination of the cooling process was studied which reveals that the temperature of the medium drops from 37°C to 29°C approximately over four and a half hours, providing valuable insight into the underlying phenomenon. Figure 4 displays the cooling process dynamics within the system, illuminating an in-depth temperature-time relationship during the cooling phase. Unlike the heating process, the data indicated a gradual reduction in temperature from the initial 37°C to 29°C. This observed cooling pattern provides valuable insights into the efficiency and dynamics of the cooling mechanism.
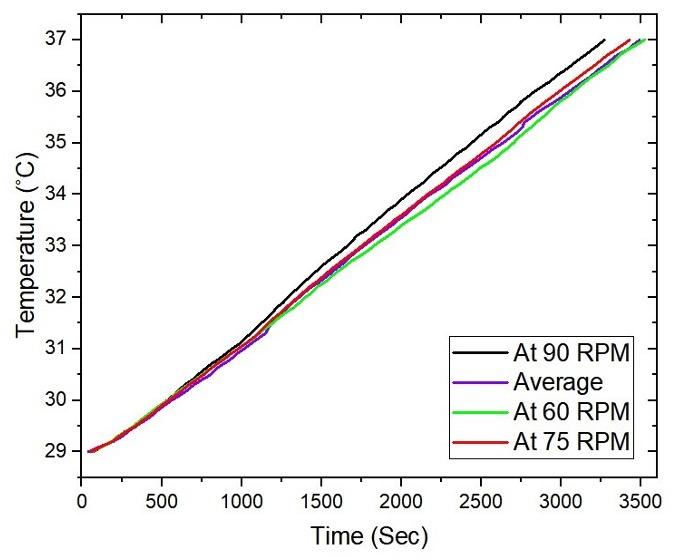
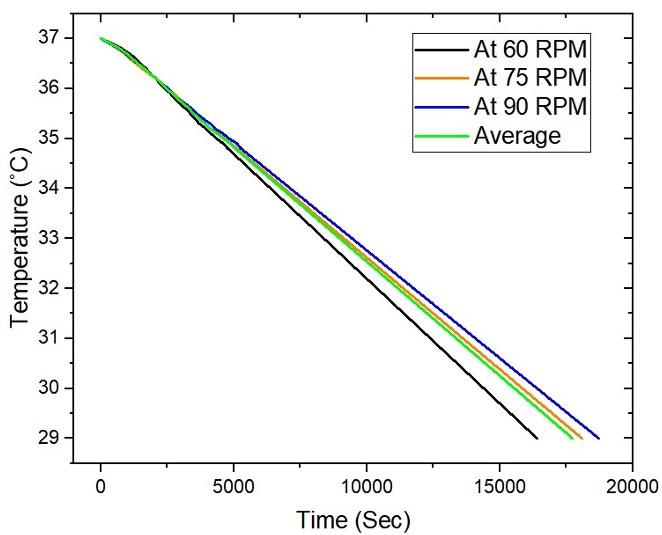
Streamline of working fluid
The Comsol Multiphysics v6.0 software was utilized to conduct a thorough simulation of the model under consideration. The simulation aimed to analyze the heated water flow around the growth chamber within the water jacket. The simulation results were presented through colored streamlines in Figure 5. The illustration shows uneven water flow distribution around the jacketed system. Streamlines do not directly flow from the inlet to the outlet line, but circulate beneath the inlet, causing a complex flow pattern. Then the streamlines follow the side walls to the lower jacket and recirculate. The absence of streamlines navigating directly from the inlet to the outlet through the lower jacket indicates a distinct flow behavior. The streamlines exiting the system are mainly on the upper side, while the circulation streamlines are on the lower side, creating turbulence. Such type of flow ensures the good distribution of temperature in a growth chamber during water circulation [38] which is crucial for maintaining an ideal environment within the chamber, ensuring all areas receive equal and regulated temperatures and producing an optimum growth of the bacterial culture.
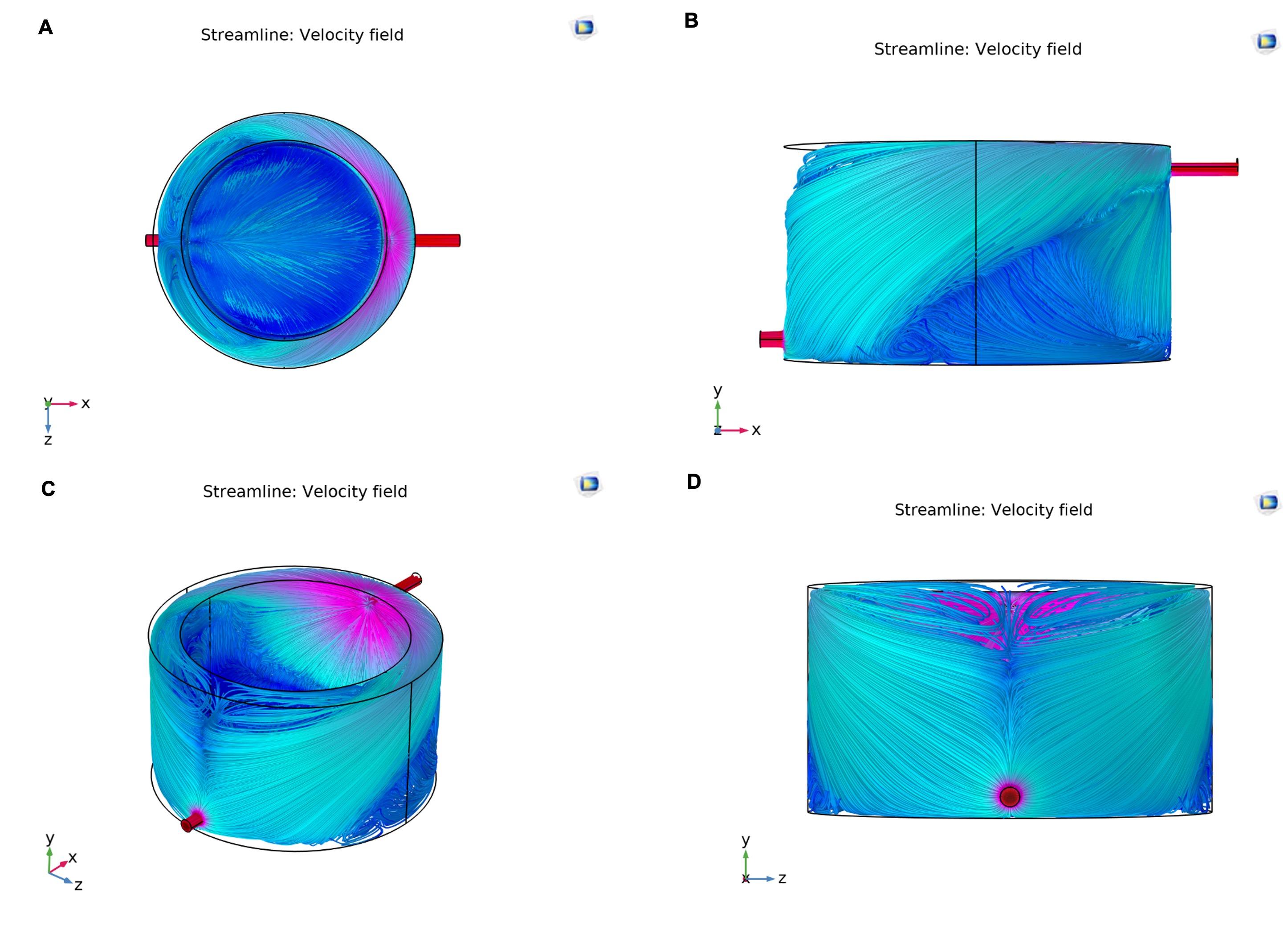
The growth rate of bacterial consortia
Figure 6A depicts the growth of all four consortia, A, B, C, and D in media containing both tryptic soya broth (5g) and yeast extract (5g). The OD values increased almost linearly from 0.25-0.5 to 1.75-2.2 throughout 12 to 48 hours of culture. From 48 to 60 hours, the growth rate significantly increased to 3 OD-3.5 OD. However, after 60 hours until 72 hours, the growth rate slows down and shows a horizontal trend with a growth rate (OD ranges 3.5~4.0). The Figure 6B shows the growth of the consortia, A, B, C, and D in media containing only tryptic soya broth (5g). The OD values exhibited a steady increase from 0.25-0.5 to 0.75-1.25 during a period of 12 to 36 hours. Following 48 hours, the growth rate increased significantly to 1.75-2.0. However, after 60 hours, the growth rate accelerated rapidly, and the trend showed a vertical increase with a growth rate ranging from an optical density (OD) value 3.25 to 3.75. On the other hand, Figure 6C demonstrates the growth of the consortia, A, B, C, and D in media containing only yeast extract (5g). The OD values showed a nearly linear increase over the entire growth period. The growth rate of consortia A, B, C, and D has been observed to increase OD value from 0.25-0.5 to 1.48-1.75 throughout the period of 12 to 48 hours. After 60 hours, the growth rate was observed OD value from 1.78 to 2.4. After 72 hours, the growth rate showed a range of OD value from 2.78 to 3.5. The consortia, A, B, C, and D in a medium containing no yeast extract and no tryptic soya broth showed a minimal growth ranging OD value from 1.75 - 2.25 (Figure 6D).
Using a combination of tryptic soya broth (5g) and yeast extract (5g) in a 25-litre sample, the growth rate of Enterobacter sp. strain HSTU-ASh6 was observed (Figure 6E). The OD value increased from 0.25 to 3.75 throughout 12 to 72 hours. The growth rate was found to be highly accelerated after 36 hours.
Overall, consortia of endophytic bacteria grown in media containing TSB and YE exhibited robust growth over the incubation period. In contrast, when either TSB or YE was absent from the medium a noticeable reduction in growth rate was observed for all consortia. The absence of both TSB and YE led to a marked decline in growth across all consortia, indicating the essential role of these components in bacterial proliferation. Further optimization experiments revealed that while both TSB and YE contributed to optimal growth, YE supplementation can be minimized without significantly compromising growth rates. Consortia grown without YE exhibited slightly reduced growth rates compared to those grown with both TSB and YE, but the difference was not substantial, suggesting that YE supplementation can be omitted to reduce production costs while still maintaining the satisfactory growth performance of the consortia. Interestingly, when a tomato plant growth-promoting endophytic bacteria, Enterobacter sp. strain HSTU-ASh6 was cultured in the bacterial growth chamber for 72 hours in presence of both TSB and YE, the high growth rate of the strain (Figure 6E) was noted like other consortia A, B, C and D (Figure 6A-D).
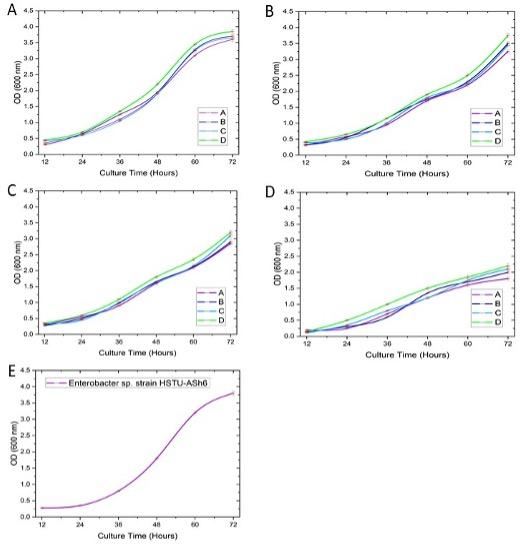
Plant growth promotion as a biofertilizer
As shown in Table 2 and Figure 7, the rice plants' height, root length, and number of tillers were all improved by the bacterial consortia-A treatment. Notably, compared to all other treatments, the number of tillers at 70% urea reduction (Treatment B) linked to the application of biofertilizer consortia A was noticeably higher (Table 2). In a similar vein, the rice plants in treatment A had the longest roots. On the other hand, treatment E was determined to have the tallest plants. These findings suggested that even in the reduction of urea fertilizer, the biofertilizer potential bacterial consortia A considerably accelerated the morphological features of rice plants associated with yields.
Table 2. Rice plants (var. BRRI dhan28) morphological traits in response to the biofertilizer consortia amplified in the newly developed fermentor.
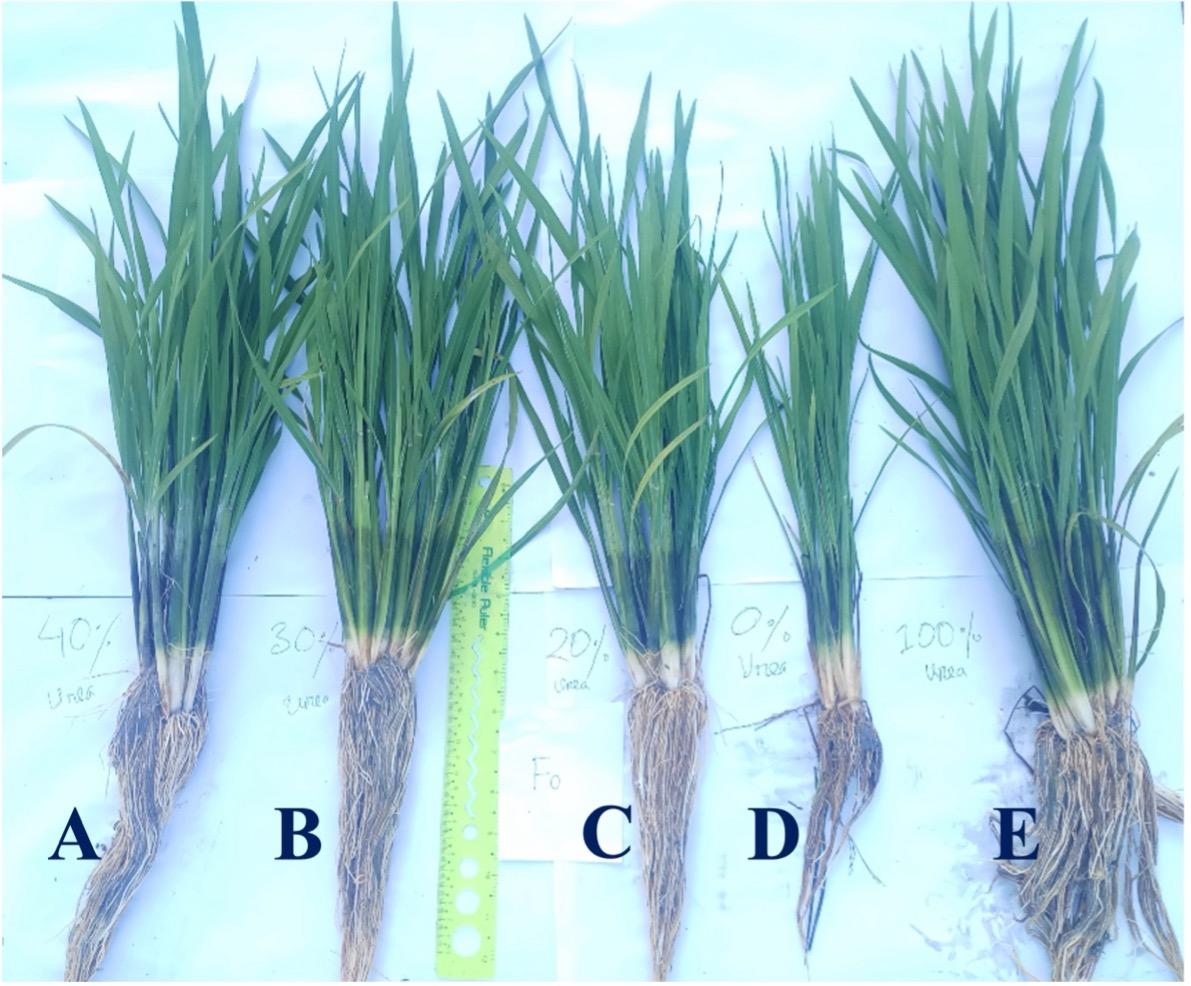
DISCUSSION
The invention of an efficient reliable fermentor with growth chambers equipped with heating and rotary motion pertains to enhanced biofertilizer production (consortia of endophytic bacteria). Biofertilizer growth chambers are devices used in the fields of biochemistry, molecular biology, bacteriology, and organic farming [38]. Continuous motion not only prevents precipitation but also ensures the circulation of solvents over all the regions of test samples, which ensures better mixing [40]. Production of bacteria in large quantities can be difficult and expensive, due to the size and cost of currently available growth systems including the culture media, as well as the availability of such systems primarily in first-world countries and the need to transport such currently available systems to other regions of the world. Therefore, a need exists for a fermentor for bio-fertilizer production that can provide heating and rotary motion in controlled conditions while remaining cost-effective and portable. Thus, to meet this challenge an affordable fermentor is well desired.
By monitoring the temperature directly from the bacterial growth chamber and heating the water in the heating chamber using an induction water heater, we can ensure that the temperature remains consistent throughout the growth process. However, slight variations have been seen due to the differences in the agitation (rpm) of the propeller. The variations in the rpm have a direct impact on the convection on the surface of the culture, leading to slight temperature variations [41]. Again, this finding is of utmost importance as it underscores the fact that the culture medium within the growth chamber can maintain its stability even in the event of a brief power disruption as the cooling process takes a very long time. This serves to highlight the prototype’s resilience and its capacity to endure minor disturbances with minimal impact. In addition, the capacity to distribute temperature uniformly is highly desirable for uniform bacterial growth. Indeed, we demonstrated that the prototype is reliable, and functioning efficiently. Several devices like ours use water jacket heating systems for a specific purpose, including the fermentation tank improvement structure, convenient for fixed stirring devices [42]; a dry powder composite coating prepared [43]; and pasteurizing apparatus [44].
A similar concept of fermentor illustrated by Yang [45] uses a water jacket for uniform heat distribution to the inner chamber. However, it does not include any integrated heating source, nor does it include a pump that is fluidly connected to the outer chamber to circulate the working fluid between the heater and the outer chamber. If we want to control the temperature of the growth chamber proposed by Yang, an externally heat-controlled water flow must be introduced. Furthermore, Yang’s model includes a complex set of mixing rods, which makes the system more difficult to manufacture, increasing the manufacturing cost and making it unaffordable for the farmers. On the other hand, the agitator used in our concept is designed in such a way that it can mix the bacterial consortium easily, an essential property deals with different types of husks of the members of the consortium, that which makes the liquid thicker. Our prototype fermentor uses a simple single motor-controlled propeller that makes it easier to build. While Yang’s Model showed the inlet and outlet of the working fluid are placed on the same side which may not result in a good fluid flow around the growth chamber, hindering the bacterial growth, the prototype presented here has the inlet and outlet on the opposite side which showed a good flow of the working fluid around the growth chamber as the simulated contour shows (Figure 5). Furthermore, our prototype demonstrates how effectively plant growth-promoting bacteria can be grown to accelerate agricultural productivity.
The system can be further modified to its range of production and temperature control. Recently, some other cost-effective concepts have been deployed to make fermentors for large-scale biofertilizer production [46] with a limit of 3.8 L biofertilizer per cycle, whereas our prototype has a capacity of 25 L per cycle with thermal stability under a power disruption. These characteristics of our compact and unique fermentor provide a design for the first-ever reported. Hence, we have patented the design under the United States Patent and Trademark Office (USPTO) [33]. The results of our study emphasized the critical role of tryptic soya broth (TSB) and yeast extract (YE) in promoting the growth of endophytic bacterial consortium in the prototype fermentor. Both single endophytic strains and consortia of different strains can adopt the growth media in the growth chamber without losing their properties. The observed robust growth of consortia in media containing both TSB and YE highlights the synergistic effects of these components on bacterial proliferation. TSB provides essential nutrients, including peptides, amino acids, and carbohydrates, while YE supplies vitamins, minerals, and growth factors necessary for bacterial growth. The combination of these components creates an optimal milieu for bacterial growth, enabling consortia to thrive and proliferate as observed [29, 30]. Furthermore, our optimization experiments revealed that YE supplementation can be minimized without significantly compromising growth rates. Consortia grown without YE exhibited slightly reduced growth rates compared to those grown with both TSB and YE, indicating that YE supplementation may be partially expendable for cost-saving purposes. This finding is particularly relevant for large-scale production efforts of biofertilizers, where reducing production costs is a priority. In the future, it is anticipated that research efforts will be directed toward refining media formulations to achieve optimal growth performance. This will involve exploring alternative nutrient sources to further enhance growth and simultaneously reduce production costs, helping sustainable organic farming.
The present study revealed a cheap formulation of growth medium can be utilized for the amplification of biofertilizer production using the newly developed prototype fermentor. This invention helps local farmers or industries to set up a sustainable technology for large-scale biofertilizer production cheaply, enhancing the crop’s yields.
The consortia (A-D) were reported to increase the rice (BRRI-dhan28) production in field conditions by 28-35% [29]. The production of tomatoes in field conditions increased 2.5-fold when treated with the single endophytic strain Enterobacter sp. HSTU-ASh6 [30]. Both rice and tomato production were increased in the presence of a 70% reduction of the recommended doses of urea and no pesticides [29]. Therefore, amplification of the bacterial strains (biofertilizers) in the novel fermentor with a cheap growth medium provided opportunities for developing sustainable organic agricultural practices. Using this technology, we have conducted several crop and vegetable field trials with various sizes of endophytic consortia that can replace numerous amounts of agrochemicals, e.g., phosphate, urea, pesticides, and increase biofunctional compounds (data unpublished). Therefore, the study provides new insights for researchers and industrialists regarding biofertilizers to ensure the sustainable development of organic agriculture and improve farming by producing hazard-free crops. The consortia A amplified in the biogrowth chamber showed noticeable improvements in the rice plant’s morphological traits (Table 1 and Figure 7) related to yields, which indicated that the biogrowth chamber created an optimal environment for the production as an endophytic bacterial-based biofertilizer.
CONCLUSIONS
This study explored a newly designed fermentor (bacterial growth chamber) as well as the newly discovered affordable medium for the production of biofertilizers for environmentally friendly organic farming. The growth medium is significantly cheaper than traditional media used for large-scale cultures of bacteria. Furthermore, the prototype fermentor has been demonstrated to be sustainable, as it uses waste products from the food industry, such as poultry feed and corn flour. We have successfully demonstrated that this new medium is used to culture a variety of next-generation plant growth-promoting bacteria in the consortium that can be used as biofertilizers for sustainable farming. This new, affordable growth medium has the potential to revolutionize the production of bacterial biofertilizers, making it more affordable and accessible to farmers around the world. The bacterial growth chamber reported in this paper is designed to provide an improved environment to produce biofertilizers. One of the main advantages of this fermentor is its ability to maintain a stable thermal environment during a prolonged power disruption, which is important for steady bacterial growth. The chamber is also designed to provide improved sterile conditions, reducing the risk of contamination and ensuring the purity of the final product. Another key feature of this prototype fermentor is its seamless operation, ensuring the growth process can proceed without interruption, allowing for efficient and consistent production of biofertilizers. Furthermore, the chamber is simple and easy to fabricate, with a low cost of approximately US $250, making it accessible and affordable for a wide range of users, including small-scale farmers. In particular, the rice plants morphological traits improvement with bacterial consortia application might be a milestone in future research for farmers practice for sustainable crop cultivation.
ACKNOWLEDGMENTS
This research was partially funded by grants from the Institute of Research and Training (IRT)-Hajee Mohammad Danesh Science and Technology University, Dinajpur, Bangladesh. The fermentor invention was patented by the United States Patent and Trademark Office (title ‘Biogrowth chamber with rotary motion and heating system and related methods’ Application number: 18/409771, priority date: January 10, 2024), Patent number US12,084,644B1 (https://patentcenter.uspto.gov/applications/18409771).
AUTHOR CONTRIBUTIONS
TA: Invention, Methodology, Investigation, Analysis, Writing; MSR: Invention, Methodology, Investigation, Analysis, Design and Simulation, Writing and Editing; R: Methodology, Writing; SG: Consulting, Review and Editing; MMH: Data Analysis; MAH: Invention, Conceptualization, Supervision, Project administration, Writing, Review and Editing; AR: Invention, Review and Editing. All authors have approved the final version.
CONFLICTS OF INTEREST
There is no conflict of interest among the authors.
References
- [1]Duro JA, Lauk C, et al. Global inequalities in food consumption, cropland demand and land-use efficiency: A decomposition analysis. Global Environmental Change. 2020;64:102124.
- [2]UN. United nations world population report. 2023.
- [3]van Dijk M, Morley T, et al. A meta-analysis of projected global food demand and population at risk of hunger for the period 2010–2050. Nature Food. 2021;2:494-501.
- [4]Fukase E, Martin W. Economic growth, convergence, and world food demand and supply. World Development. 2020;132:104954.
- [5]Pahalvi HN, Rafiya L, et al. Chemical fertilizers and their impact on soil health. Microbiota and Biofertilizers, Vol 2: Ecofriendly tools for reclamation of degraded soil environs. 2021:1-20.
- [6]Rashmi I, Roy T, et al. Organic and inorganic fertilizer contaminants in agriculture: Impact on soil and water resources. Contaminants in Agriculture: Sources, Impacts and Management. 2020:3-41.
- [7]Planning Mo. Foreign trade stastics of bangladesh. 2022-23.
- [8]Walker DJ, Clemente R, et al. Contrasting effects of manure and compost on soil ph, heavy metal availability and growth of chenopodium album l. In a soil contaminated by pyritic mine waste. Chemosphere. 2004;57:215-24.
- [9]Amoo AE, Enagbonma BJ, et al. Biofertilizer: An eco-friendly approach for sustainable crop production. Food Security and Safety: African Perspectives. 2021:647-69.
- [10]Verma DK, Pandey AK, et al. Plant growth-promoting rhizobacteria: An eco-friendly approach for sustainable agriculture and improved crop production. Microbiology for sustainable agriculture, soil health, and environmental protection: Apple Academic Press; 2019. p. 3-80.
- [11]Ha-Tran DM, Nguyen TTM, et al. Roles of plant growth-promoting rhizobacteria (pgpr) in stimulating salinity stress defense in plants: A review. International Journal of Molecular Sciences. 2021;22:3154.
- [12]Enebe MC, Babalola OO. The influence of plant growth-promoting rhizobacteria in plant tolerance to abiotic stress: A survival strategy. Applied microbiology and biotechnology. 2018;102:7821-35.
- [13]Etesami H, Adl SM. Plant growth-promoting rhizobacteria (pgpr) and their action mechanisms in availability of nutrients to plants. Phyto-Microbiome in stress regulation. 2020:147-203.
- [14]Nagrale D, Chaurasia A, et al. Pgpr: The treasure of multifarious beneficial microorganisms for nutrient mobilization, pest biocontrol and plant growth promotion in field crops. World Journal of Microbiology and Biotechnology. 2023;39:100.
- [15]Di Benedetto NA, Corbo MR, et al. The role of plant growth promoting bacteria in improving nitrogen use efficiency for sustainable crop production: A focus on wheat. AIMS microbiology. 2017;3:413.
- [16]Verma M, Mishra J, et al. Plant growth-promoting rhizobacteria: Diversity and applications. Environmental biotechnology: for sustainable future. 2019:129-73.
- [17]Chauhan H, Bagyaraj D, et al. Novel plant growth promoting rhizobacteria—prospects and potential. Applied Soil Ecology. 2015;95:38-53.
- [18]Khan N, Bano A, et al. Crosstalk amongst phytohormones from planta and pgpr under biotic and abiotic stresses. Plant Growth Regulation. 2020;90:189-203.
- [19]Bhat M, Rasool R, et al. Plant growth promoting rhizobacteria (pgpr) for sustainable and eco-friendly agriculture. Acta Sci Agric. 2019;3:23-5.
- [20]Martínez-Viveros O, Jorquera MA, et al. Mechanisms and practical considerations involved in plant growth promotion by rhizobacteria. Journal of soil science and plant nutrition. 2010;10:293-319.
- [21]Etesami H. Plant–microbe interactions in plants and stress tolerance. Plant life under changing environment: Elsevier; 2020. p. 355-96.
- [22]Asghari B, Khademian R, et al. Plant growth promoting rhizobacteria (pgpr) confer drought resistance and stimulate biosynthesis of secondary metabolites in pennyroyal (mentha pulegium l.) under water shortage condition. Scientia Horticulturae. 2020;263:109132.
- [23]Abhilash P, Dubey RK, et al. Plant growth-promoting microorganisms for environmental sustainability. Trends in Biotechnology. 2016;34:847-50.
- [24]Ojkic N, Lilja E, et al. A roadblock-and-kill model explains the dynamical response to the DNA-targeting antibiotic ciprofloxacin. BioRxiv. 2019:791145.
- [25]Maier RM, Pepper IL. Bacterial growth. Environmental microbiology: Elsevier; 2015. p. 37-56.
- [26]Pörtner R, Nagel-Heyer S, et al. Bioreactor design for tissue engineering. Journal of bioscience and bioengineering. 2005;100:235-45.
- [27]Lara AR, Galindo E, et al. Living with heterogeneities in bioreactors: Understanding the effects of environmental gradients on cells. Mol Biotechnol. 2006;34:355-81.
- [28]Vassilev N, Vassileva M, et al. Unexploited potential of some biotechnological techniques for biofertilizer production and formulation. Applied microbiology and biotechnology. 2015;99:4983-96.
- [29]Prodhan MY, Rahman MB, et al. Characterization of growth-promoting activities of consortia of chlorpyrifos mineralizing endophytic bacteria naturally harboring in rice plants—a potential bio-stimulant to develop a safe and sustainable agriculture. Microorganisms. 2023;11:1821.
- [30]Haque MA, Hossain MS, et al. Unveiling chlorpyrifos mineralizing and tomato plant-growth activities of enterobacter sp. Strain hstu-ash6 using biochemical tests, field experiments, genomics, and in silico analyses. Frontiers in Microbiology. 2022;13:1060554.
- [31]Baddoo N. Stainless steel in construction: A review of research, applications, challenges and opportunities. Journal of constructional steel research. 2008;64:1199-206.
- [32]Tran T, Kannoorpatti K, et al. A study of bacteria adhesion and microbial corrosion on different stainless steels in environment containing desulfovibrio vulgaris. Royal Society open science. 2021;8:201577.
- [33]Haque MA. Biogrowth chamber with rotary motion and heating system and related methods’ US patent 2024, Patent number US12,084,644B1 (https://patentcenter.uspto.gov/applications/18409771)
- [34]Janna WS. Engineering heat transfer: CRC press; 2018.
- [35]Sidebotham G. Heat transfer modes: Conduction, convection, and radiation. In: Sidebotham G, editor. Heat transfer modeling: An inductive approach. Cham: Springer International Publishing; 2015. p. 61-93.
- [36]Cengel Y, Cimbala J, et al. Ebook: Fundamentals of thermal-fluid sciences (si units): McGraw Hill; 2012.
- [37]Moon S, Ham S, et al. Temperature matters: Bacterial response to temperature change. Journal of Microbiology. 2023;61:343-57.
- [38]Patil PM, Yadav AP, et al. Comparative study between heat transfer through laminar flow and turbulent flow. Int J Innov Res Sci Eng Technol. 2015;4:2223-6.
- [39]Mahanty T, Bhattacharjee S, et al. Biofertilizers: A potential approach for sustainable agriculture development. Environmental Science and Pollution Research. 2017;24:3315-35.
- [40]Yunus MU, Silas K, et al. A review of biofertilizer production: Bioreactor, feedstocks and kinetics. 2022.
- [41]Agrawal S, Simon T, et al. An experimental study on the effects of agitation on convective heat transfer. International Journal of Heat and Mass Transfer. 2015;90:302-13.
- [42]Liao C-b. Fermentation tank improvement structure convenient for fixd stirring device China2016.
- [43]Li W-j. A dry powder composite coating prepared by the preparation method and its device. China2020.
- [44]J.W. Ladd HBW. Pasteurizing apparatus. USA1932.
- [45]Yang Z, Qu. A multi-bacteria fusion symbiotic fermentation technique for disease resistance and promoting growth. China2021.
- [46]Manzano-Gómez LA, Rincón-Rosales R, et al. Cost-effective cultivation of native pgpb sinorhizobium strains in a homemade bioreactor for enhanced plant growth. Bioengineering. 2023;10:960.