Plant improvement and metabolite production in Cannabis sativa: Recent biotechnological advances
Abstract
The Cannabis sativa plant is an excellent source of metabolites, fiber, and medicinal properties. Phytocannabinoids are the secondary metabolites naturally derived from Cannabis plant species. These metabolites are promising and can be used in producing phytomedicine or plant-based therapeutics. However, many of these compounds are produced in low quantities across different Cannabis species. To solve this limitation, in vitro, biotechnological methods offer promising solutions for enhancing the production of secondary metabolites in Cannabis. This review highlights the biotechnological approaches for enhancing Cannabis secondary metabolite production through in vitro plant improvement techniques such as plant regeneration, elicitor-responsive metabolite induction, polyploidy manipulation, protoplast culture, bioreactor-based hairy root culture, genetic transformation, and genome editing. These biotechnological approaches might be useful for improving Cannabis plants and increasing plant capacity to produce potential metabolites. These phytochemical and bioactive compounds found in Cannabis species could be used as alternative resources for pharmaceutical and industrial production.
INTRODUCTION
Cannabis sativa L. is a high-value multipurpose plant. In early societies, this plant was used for medicinal benefits, and recreational elements, as a source of food additives, cosmetics, paper, bioenergy, and textiles [1]. Additionally, Cannabis possesses pharmacological benefits, such as anti-cancer, anti-inflammatory, antispastic, anticonvulsant, anti-pruritic, and anti-psychotic elements [2]. It is also used for ornamental and landscaping purposes [3]. Phytocannabinoids are the primary secondary metabolites found in Cannabis, and are primarily formed in the glandular trichomes of female Cannabis flowers [4]. Over 150 phytocannabinoids have been identified in Cannabis plants [5]. Research associated with signalling pathways of phytocannabinoids leads to the identification of cannabinoid receptors and their endogenous ligands [6-9]. These receptors have been found in various cellular compartments, which are associated with brain disorders, metabolic pathways, and immune function [8]. Some cannabinoids are used for potential therapeutic use in treating COVID-19 [10-12]. The plant containing cannabinoids, terpenes, and phenolic compounds is increased due to its pharmacological applications [13, 14]. Breeding of Cannabis cultivars with specific secondary metabolite profiles for medicinal purposes is a slow process due to dioecy and regulatory constraints [15, 16]. Therefore, alternative strategies for the rapid and efficient production of minor cannabinoids (CBG, CBN, CBC, and THCV), which are produced in lower quantities than major cannabinoids (THCA, CBDA), are of particular research interest [17].
In vitro tissue culture methods, including callus, cell culture, de novo regeneration, hairy root culture, and protoplast culture, are essential for micropropagation [18], and genetic engineering techniques (e.g., Agrobacterium-mediated gene transformation, A. rhizogenes-mediated hairy root cultures, etc.) as well as polyploidy induction can be used to improve traits with producing secondary metabolites in plants [19]. These traditional methods can alter secondary metabolite production, the CRISPR/Cas9 system is one of the most significant approaches for faster genetic manipulation in Cannabis, especially in overcoming in vitro recalcitrance [20, 21]. In this updated study, we focused on advances of Cannabis biotechnology approaches related to plant improvement and potential metabolite production, which will open a new era of Cannabis research.
BIOSYNTHETIC PATHWAYS FOR METABOLITES PRODUCTION
Two main pathways are involved in the synthesis of cannabinoids in Cannabis plants. The polyketide pathway that produces olivetolic acid (OLA), and the plastidal 2-C-methyl-d-erythritol 4-phosphate (MEP) pathway that synthesises geranyl diphosphate (GPP). OLA originates from hexanoyl-CoA through aldol condensation with three molecules of malonyl-CoA by a polyketide synthase (PKS) enzyme and an olivetolic acid cyclase (OAC) through polyketide pathway (PKP) (Figure 1). The alkylation of OLA with GPP by geranyl pyrophosphate: olivetolate geranyl transferase forms the central precursor CBGA. Oxidocyclases such as THCA synthase (THCAS), CBDA synthase (CBDAS), and CBCA synthase (CBCAS) then contribute to the diversity of cannabinoids (Figure 1) [13].
Terpenes, vital compounds in plant biology, are synthesised through two distinct pathways. The cytosolic mevalonic acid (MVA) pathway produces sesqui- and triterpenes, while the plastid-localized MEP pathway synthesises mono-, di-, and tetraterpenes. These pathways start from acetyl-coenzyme A and pyruvate and d-glyceraldehyde-3-phosphate, respectively, leading to the production of isopentenyl diphosphate (IPP) and dimethylallyl diphosphate (DMAPP) as the end products. In the cytosol, farnesyl diphosphate synthase (FPS) combines two molecules of IPP (C5) and one molecule of DMAPP (C5) to form FPP, a precursor for sesquiterpenes (C15). Squalene synthase (SQS) in the endoplasmic reticulum uses two FPP to generate precursors for triterpenes and sterols. One molecule of IPP and one molecule of DMAPP are condensed to form an intermediate precursor of monoterpenes GPP (C10) by GPP synthase (GPS) in the plastid [13] (Figure 1).
Phenolic compounds are synthesized in the cytoplasm through the phenylpropanoid pathway and transported to the vacuole or cell wall. The main classes of phenolic compounds are produced via the core phenylpropanoid pathway from phenylalanine to p-coumaroyl CoA, involving enzymes like phenylalanine-ammonia-lyase (PAL), cinnamate 4-hydroxylase (C4H, a cytochrome P450) and 4-coumarate-CoA ligase (4CL). Additional pathways lead to the formation of simple esters, lignins and lignans, flavonoids, coumarins, and stilbenes. The flavonoid pathway starts with condensing p-coumaroyl CoA and malonyl-CoA (Figure 1), producing various flavonols like kaempferol and quercetin. In the next step, naringenin is converted to apigenin and luteolin, which are precursors of cannflavins (Figure 1) [13].
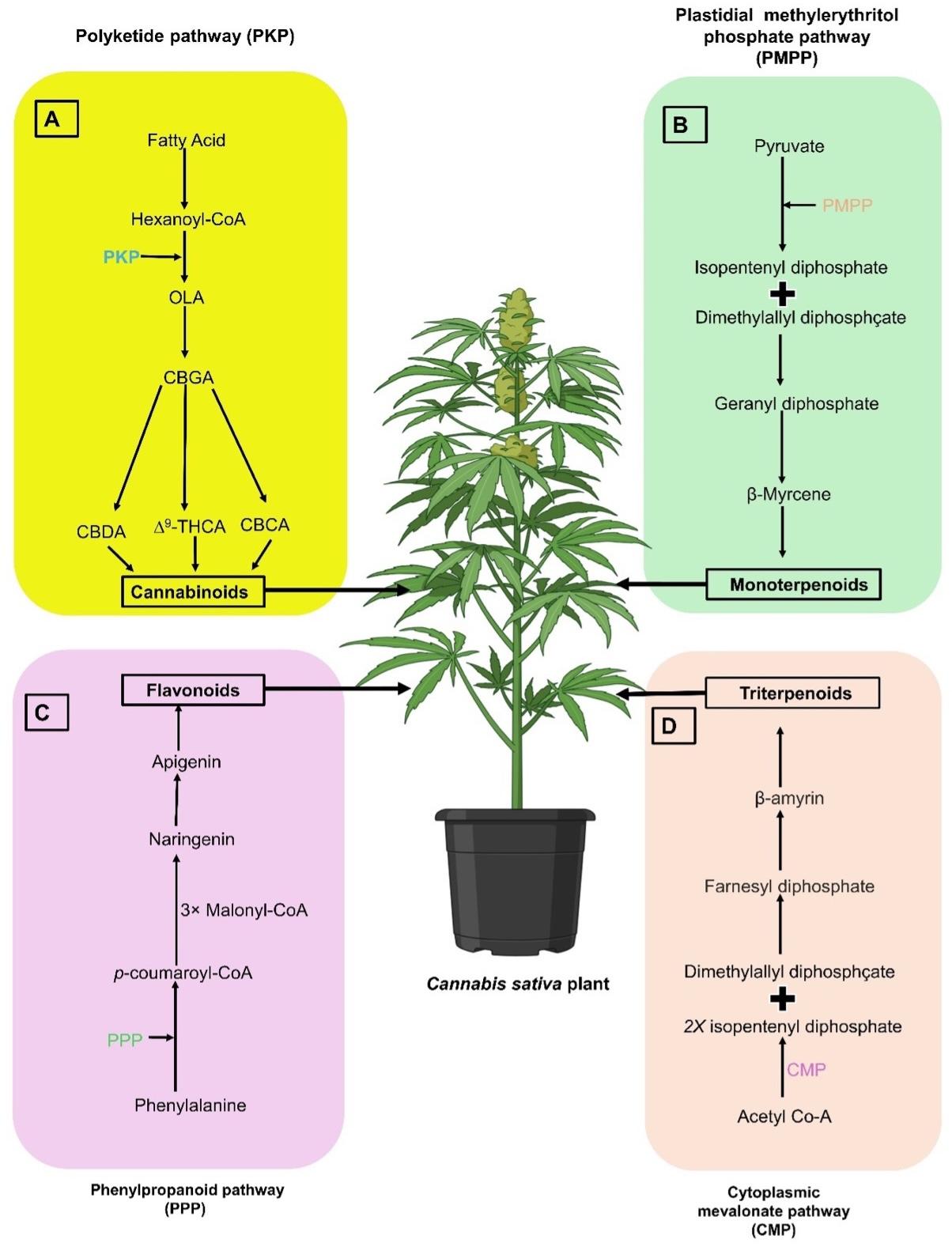
LIMITATIONS OF CONVENTIONAL BREEDING
C. sativa has nine pairs of autosomes and a pair of sex chromosomes (XY for males and XX for females). The determination of sex in Cannabis is influenced by several environmental factors. Sex chromosomes in Cannabis are not well understood, making sex determination a challenge in breeding new varieties. Dioecy in Cannabis is controlled by two specific genes at linked loci. Sex can only be determined at the beginning of flowering when male and female flowers are visible. So Dioecy caused heterogeneity is a crucial constraint in conventional breeding [21,22].
General Cannabis and other drug-type varieties have historically been bred through mass selection to improve quality traits like fiber, oil, and cannabinoid content. Early genetic improvement efforts faced challenges in avoiding high THC hemp genotypes and obtaining uniform medical genotypes. Recent advancements in breeding involve controlled mating of selected individuals from different landraces and cultivars. Synthetic varieties are created through open pollination using multiple female and male plants propagated via cuttings. High THC-type Cannabis, being dioecious, is often vegetatively propagated indoors to maintain genetic purity and uniformity. Indoor cultivators typically rely on cuttings from a mother plant, but this method requires space and can lead to decreased vigor, susceptibility to pests and diseases, and potential disease transmission [21, 22].
In vitro techniques present a promising approach for large-scale production and germplasm maintenance. Tissue culture-based clean plant programs have successfully controlled plant pests, diseases, and viruses in vegetatively propagated crops and horticultural and ornamental crops. Developing an optimized in vitro method for propagating clean plants is crucial for producing genetically identical plants on a large scale, preserving genetic fidelity, and ensuring the long-term sustainability of economically significant Cannabis varieties [14, 22].
RECENT BIOTECHNOLOGICAL ADVANCES IN CANNABIS
In vitro plant growth and development
Recent advances of Cannabis biotechnology have led to improved plants with traits. Research on optimising Cannabis micropropagation has found that genotype, nutrient composition of the medium, and growth regulator supplementation significantly influence Cannabis propagation efficiency [23, 24]. Establishing procedures for iterative micropropagation is essential for producing plant material for research purposes and multiplying germplasm efficiently [25]. While MS-based media are commonly used for C. sativa micropropagation, some studies suggest that a DKW-based medium with increased levels of sulfur (~7×), calcium (~3×), and copper (10×) can enhance stage 2 explant growth over an extended period without decline [26].
Glucose has been tested as a carbon source for in vitro seed germination of industrial hemp. Still, it showed lower germination rates than sucrose, commonly used in hemp micropropagation studies. Glucose resulted in more vigorous plantlets across ten hemp genotypes, indicating an interaction between nutrient medium and carbon source for plant vigour. Maintaining a proper sugar-to-nitrate ratio is crucial for nitrogen uptake in vitro, with the highest ratios observed in DKW–glucose medium promoting vigorous growth [27].
Microbial contamination is a common issue in tissue culture due to nutrient-rich media commonly used Murashige and Skoog basal medium or its variants [28]. H2O2 is effective against microbes [29] and also plays a role in plant signaling for germination [30]. A 1% H2O2 solution promoted rapid and successful germination of various C. sativa genotypes and contamination-free seedlings as explant sources in antibiotic-free MS media [31, 32]. In vitro, Cannabis seed germination is slower than field germination due to the absence of microbes that aid seed coat digestion. Scarification or removal of the seed coat is crucial for breaking seed dormancy, promoting faster germination with sodium hypochlorite and hydrogen peroxide, and improving germination speed and frequency. Low salt concentrations enhanced seed germination rate and resulted in longer hypocotyls and radicles compared to high salt concentrations [31, 33].
The source of the explant is a crucial factor in rejuvenation practices to combat culture decline. Variability among explant types hinders the low efficiency of in vitro plant regeneration protocols for C. sativa. This variation poses a challenge for using tissue culture to improve this species. When two primordia emerge from the top of hypocotyls, they are always located on the organ's periphery and aligned in a specific way, leading to consistent plant regeneration from the same type of cells. This regeneration pattern closely resembles the regeneration area of 7 days seedling hypocotyl-derived meristems treated with ZEARIB 2.0 (mg/L) or ZEARIB 1.0 (mg/L) + NAA 0.02 (mg/L), resulting in a 66.67% response rate without any plant growth regulator application [34]. The tissue's size, type, and developmental phase influence the success of response induction. Shoots originating from the basal and near-basal regions of the plant yielded taller shoots with more nodes than those from the middle and apical portions [35]. Explant sources from basal portions yielded Cannabis plantlets characterised by shorter stature yet broader leaves, suggesting potential for in vitro rejuvenation with minimal culture degradation [35].
Hedging, a modified shoot tip culture technique, has been successfully applied in commercial horticultural crops [79]. This method involves repeated harvests to eliminate the need for replanting, saving labour and resources. In Cannabis cultivation, an in vitro hedging technique was used to promote lateral shoot regrowth by removing shoot tips after three weeks [36]. The number of shoot tips harvested over multiple cycles, especially with higher light intensity. This method can enhance axillary divisions without the use of external plant growth regulators, leading to improved photoautotrophic metabolism [35, 36]. Cuttings in the initial phases are placed in vessels with passive gas exchange under aseptic conditions, which is crucial for developing photoautotrophic micropropagation. In this technique, chlorophyllous explants are grown in CO2-rich environments. Using gas-permeable film vessels and porous substrate has proven effective for the photoautotrophic growth of plant species utilising a double-phase culture system, where shooting and rooting occur on semi-solid and liquid medium layers termed bioreactors. Such bioreactors have also been used to alter explant physiology. Rooting sponges have shown promising improvement in the aeration of the rhizosphere [53,54].
Plant cellular development encompasses the emergence of shoot primordia, apical meristems, leaf primordia, and procambium strands originating from the base of the floral explant where meristem centers develop at the junction between the filament and tepal, a phenomenon known as floral reversion [37]. The mode of floral reversion is species-specific and holds significance in plant biotechnology based on transient gene expression have revealed the potential to express transgenes in floral tissues, rendering floral reversion a valuable tool in commercial crop production and for species that can overcome challenges in genetic modification [38]. TDZ, a compound with auxin and cytokinin-like properties, enhanced callus induction in C. sativa floral tissues. Additionally, Using cytokinins, mT(metatopolin), and BAP promoted better shoot proliferation instead of callus induction from in pairs of florets rather than single florets [38].Rooting and acclimatization are challenging stages in hemp and Cannabis tissue culture. Using sodium metasilicate, AgNO3, IBA, kinetin, or 2,4-D can aid this process. Sodium metasilicate enhances foliage appearance and rooting rate. The efficiency of plantlet recovery depends on Cannabis genotype, endophytic contamination, and rooting frequency [39]. Incorporating potassium silicate as a silicon source into the growth medium demonstrated a notable reduction in hyperhydricity symptoms across three cultivars, concurrently enhancing root induction [28].
Low branching tendency and high apical dominance in fiber-type hemp result in a low multiplication rate and difficulties in initiating multi-shoot cultures [24, 40]. Apical dominance is a plant phenomenon where the main shoot inhibits the growth of axillary buds [41] and it is controlled by a complex network of hormones, including auxin flow from lateral buds [42-44] and hormonal interactions between strigolactones and cytokinins play a role in bud outgrowth [45-47]. The outgrowth of axillary buds depends on the ratio of these plant hormones [44, 46, 48]. The N-1-naphtylphtalamic acid, and 2,3,5-triiodobenzoic acid (TIBA) are known as auxin polar transport inhibitors that can break apical dominance and enhance shoot regeneration. These findings are valuable for the micropropagation of challenging industrial hemp varieties [49].
One approach to enhancing the rate of shoot multiplication in vitro is to interfere with apical dominance, which is often significant in Cannabis due to high levels of endogenous auxin. A promising compound for this purpose is α-(2-oxo-2-phenylethyl)-1H-indole-3-acetic acid (PEO-IAA), known for its solid anti-auxin activity. Auxin is a crucial plant hormone that regulates apical dominance through a short but universal signalling pathway that swiftly shifts between repressing and activating gene transcription by degrading transcriptional repressors dependent on auxin. At elevated auxin levels, auxin is believed to enhance binding between TIR1/AFB and AUX/IAA repressors, forming the core of the auxin signalling pathway long with auxin-responsive (ARF) transcription factors. When auxin levels drop, AUX/IAA repressors become more stable, increasing their interaction with ARF to suppress transcriptional activities. These auxin signalling cascades govern various cellular processes by activating or repressing distinct gene groups, including cell growth, expansion, and differentiation, which remain unclear. RT-qPCR analysis demonstrated that PEO-IAA in the culture medium affected the relative gene expression of targeted metabolites biosynthetic genes (OAC, CBCA, CBDA, THCA) and the levels of essential cannabinoids (CBCA, CBDA, CBC, ∆9-THCA, and ∆9-THC) [50, 51].
Cannabis is typically a short-day plant, but auto-flowering genotypes are available. Short-day plants need a long dark period to induce flowering, which is more crucial than the light period [52]. Most Cannabis micropropagation is done under long-day photoperiods (16.0–18.0 hrs per day), keeping plants in a vegetative state. However, initial observations indicate that some genotypes flower in vitro, even under long days, showing the potential for in vitro flower development in Cannabis. Understanding genotype-specific photoperiods can optimize growth by providing the ideal conditions for photosynthesis. In a study, most in vitro flowering was seen under 12.0- and 13.2-hour treatments. In vitro flowering shows an efficient way to study floral and/or seed seed-based organ development and a new route of secondary metabolism in Cannabis [53].
In vitro culture can lead to genetic variation due to medium composition and plant growth regulators. The stability of regenerated plants is also a concern due to the frequent manipulation of different factors during micropropagation. To ensure the quality of plant material, genetic homogeneity was assessed using SSR markers in two Cannabis sativa varieties rich in CBD and CBG. This result suggested that in vitro multiplication protocols are suitable for clonal mass propagation with expected genetic stability [54]. Overall discussions from the above, it can be concluded that complex interaction between several factors can make the in vitro growth and development of Cannabis sativa successful or unsuccessful. What they have yet to do is insufficient to claim their robustness. More focus should be given to multi-genotype trials for such outcomes.
In vitro recalcitrance of Cannabis species
Various factors, such as plant hormones, nutrient composition, physical conditions, plant growth phase, explant nature, and season, are manipulated to induce organogenesis from the callus. However, despite these efforts, certain plant species are highly recalcitrant to tissue culture and may not induce organogenesis. The process of plant recalcitrance can inhibit plant organelle to response tissue manipulation [55].
A three-part regeneration system (callus-shooting-rooting) was previously successful in leaf explants from a high-THC genotype. It showed callogenesis on Murashige & Skoog (MS) medium with 0.5 μM of α-naphthaleneacetic acid (NAA) and 1.0 μM thidiazuron (TDZ) (Callus medium). Regeneration was achieved by transferring cultures to MS medium with 0.5 μM TDZ (shooting medium), resulting in a 96.6% response rate with an average of 12.3 shoots per culture. Rooting was successful on a medium with half-strength MS salts and 2.5 μM indole-3-butyric acid (IBA) (rooting medium). A multi-genotype study confirmed the effectiveness of the callus medium, achieving a 100% response rate across drug-type genotypes. These results highlight the importance of using multiple genotypes in vitro study with sufficient replication [20]. Cannabis calli can be classified into two main groups, embryogenic and non-embryogenic, based on their morphology. The non-embryogenic callus can be friable or compact with no organ regeneration potential. Although not suitable for regeneration, it can be valuable for secondary metabolite production [56].
Embryogenic callus formation in Cannabis sativa is triggered by exogenous PGRs in tissue culture media. This process originates from cells with similar genetic backgrounds and morphologies. From a recent comparative transcriptomic study, upregulation of receptor-like proteins (RLPs) in embryogenic callus proved exogenous hormones play a vital role in in vitro regeneration. Most of the 12.4% of upregulated gene product properties were associated with cellular components. Cell wall modifications are essential for maintaining signalling factor transformation through a cellular connection. Various cell wall-modifying enzymes, cell wall-related signalling transduction genes, transcription factors interacting with chromatin modifiers (HDAC, CRF, etc.), Polycomb repressive complex (PRCs), and phytohormone-related genes differentially expressed in different types of calli, also highlighted the importance of PGRs in callogenesis. Studies have shown that imbalanced expression of auxin-cytokinin signalling pathway genes can lead to the conversion of non-embryogenic callus to embryogenic callus [56]. The expression levels of genes involved in the cytokinin-dependent pathway resulted in embryogenic callus formation. In contrast, the repression of genes in the auxin-dependent pathway suppressed somatic embryogenesis. This suggests that the recalcitrant nature of Cannabis may be due to the repression of auxin-dependent pathway genes. Developing embryogenic tissues in Cannabis may differ from the conventional embryogenic pathway leading to plant regeneration. In this context, CRISPR-based genome editing techniques may provide exciting solutions for Cannabis development [56].
Temporary immersion system in Cannabis sativa
In vitro, micropropagation traditionally involves maintaining numerous culture vessels with semi-solid media and transferring plant material to fresh media every 4–6 weeks due to the medium's exhaustion of nutrients. This method is labour-intensive and costly due to the use of gelling agents. To reduce costs and improve efficiency, liquid medium and bioreactor cultures, such as Temporary Immersion system (TIS) for micropropagation, have become popular alternatives, allowing for easy plantlet scaling up, simplified handling, efficient nutrient uptake, and enhanced growth rates. However, challenges such as asphyxia, hyperhydricity, and physiological disorders may arise in liquid cultures [57, 58]. Additionally, factors such as immersion frequency, liquid medium volume, number of explants, aeration, and forced ventilation are critical for optimising micropropagation using TIS [58, 59].
An efficient bioreactor approach with a ventilation system can improve photosynthesis and reduce contamination, leading to healthier shoots. Cannabis shoots in bioreactors grew with 0.5% sucrose but did not grow without sugar. CO2-enriched air can promote autotrophy. Bioreactor design influences micropropagation success. Future research could explore additional aeration in bioreactors, light intensity, light quality, Photoperiod, CO2 supplementation, and sucrose’s impact on Cannabis rooting and acclimation in a variety of Bioreactor systems [58, 59].
In vitro polyploidization
Polyploidisation induced by antimitotic agents is common in plants and can increase genetic diversity in specific plant lineages. This process often results in polyploids with unique phenotypic traits, such as larger flowers or leaves. Studies have shown that tetraploids of medicinal plants, including Cannabis, exhibit higher concentrations of active metabolites [60, 61]. Cannabis is a diploid plant with 20 chromosomes. Increasing the chromosome set through polyploidisation may enhance potency or customize cannabinoid ratios. Some studies suggest that polyploid Cannabis could have higher potency, but results vary, with some studies showing a decrease in THC levels.
The polyploidisation on drug-type Cannabis strains is not well understood. In one study, a THC/CBD balanced drug-type strain of C. sativa was treated with the herbicide oryzalin to create polyploids. The specificity of oryzalin for plant tubulins is higher [172] and is considered a more effective and less toxic alternative to colchicine. It also indicates that oryzalin is effective at over 30 times lower concentration than colchicine [150,153,173-176].
Cultured axillary bud explants were treated with various concentrations of oryzalin for 24 h, resulting in a higher number of tetraploids. Tetraploid plants exhibited larger leaves with leaves with 30% more stomata and 40% higher trichome density, critical sites for secondary metabolite production. There were observed considerable alterations in the terpene profile that influence the CBD levels in buds [60, 62]. The cannabinoid ratio is influenced by specific enzymes on chromosome 6. Different cultivars have unique enzyme variants affecting cannabinoid production.
Genome rearrangements like polyploidisation can create new enzyme combinations, leading to novel chemotypes with varied cannabinoid and terpene levels. Tetraploid sugar leaves show a 71.5% increase in terpene content due to higher trichome density. Buds also have about 30% more terpenes, indicating increased trichomes on flowers. However, the reason for the lack of a significant increase in cannabinoids with higher trichomes density remains unclear [60, 61]. Additional analysis of tetraploid individuals in Cannabis can help to determine if polyploidisation results in larger floral size. Subsequent testing is needed to assess the stability of tetraploid clones over multiple generations and whether this stability is maintained when plants are propagated from seeds [60, 63].
IMPROVEMENT OF CANNABIS SATIVA
In vitro protoplast culture of Cannabis sativa
Protoplasts are plant cells without cell walls, isolated from tissues like leaves, petals, and roots. They are totipotent and can form colonies, develop into callus, and regenerate plants. Protoplast fusion creates new cultivars with desired traits, especially in incompatible plants. Protoplasts can also be used for genetic modification, including CRISPR/Cas9-mediated genome editing. This technology allows for efficient validation of mutagenesis and DNA-free gene editing [64, 65]. Hemp is a promising candidate for new plant breeding—technologies (NPBT) like CRISPR/Cas9-mediated gene editing. One potential target is the THCA synthase gene to create hemp plants with no THC production and potentially increased production of other significant minor cannabinoids. However, applying CRISPR/Cas9 to Cannabis breeding faces challenges due to the difficulty of working with Cannabis and the lack of methods for regenerating transgenic plants. Isolating protoplasts from Cannabis plants can be complex and influenced by species, growth conditions, tissue source, pretreatment, enzymes, and buffer composition (Figure 3) [25].
Auxin and its derivatives are frequently utilised in Cannabis tissue culture. Recent Studies have linked auxin signalling in Cannabis to the development of female flowers. Protoplasts co-transformed with the DR5: GFP reporter and auxin response factors, along with a plasmid containing a p35S: RFP expression cassette, showed successful transformation in 31% of protoplasts. These transformed protoplasts exhibited a robust response to a 16 h treatment with 5 μ M indole-3-acetic acid (IAA), resulting in nearly a four-fold increase in DR5:: GFP signal [25].
Fluorescence microscopy analysis of the p35S: GFP construct showed that GFP signals of CsCBCAS, CsCBDAS, and CsTHCAS proteins were not evident as nuclear markers At ARR2-RFP. This suggests these proteins are localised in a cytoplasmic organelle outside the nucleus. However, the specific organelle where they are located remains unknown in Cannabis protoplasts. To determine the exact localisation of these enzymes, a co-expression study with cytoplasmic organelle markers for peroxisomes, mitochondria, vacuoles, and endoplasmic reticulum (ER) is required [66].
Optimising protoplast isolation from Cannabis involves a combination of digestion solutions. The best results were achieved with a protocol using 0.4M mannitol, a mix of enzymes (cellulase: macerozyme: pectolyase; 1.5%: 0.4%: 1.0), and vacuum-permeating treatment, yielding 9.7×106 viable protoplasts (g/ FLW). Enzymolysis times of 8 -16 h were optimal for high viability without over-digestion. Transient expression of GFP reached 23.2% efficiency with 30 μg plasmid, 50% PEG, 1 × 106 protoplasts, and a transfection duration of 20 min. [67, 68]. An investigation of what has been done till now is insufficient to establish a robust protocol. More studies are warranted to optimise all the factors for a thriving protoplast culture to manipulate metabolic pathway genes.
Improvement of Cannabis through genome editing
Genome editing is an efficient tool for introducing foreign genes into crops to enhance traits such as herbicide resistance, pro-vitamin A production, insect resistance, etc [69]. In Cannabis, developing gene transformation and genome editing systems can modify horticultural traits, growth morphology, and stress resistance while also enabling gene function studies. Agrobacterium involving tumor-inducing gene delivery [70] and Agrobacterium-mediated transformation is a common technique involving the transfer of genes via a plasmid containing phytohormone and opine synthase genes, which are transferred into plant cells through a type IV (pills) secretion system. Subsequently, they are incorporated into plant chromosomes, leading to gall formation. Recombinant strains can be created by engineering natural A. tumefaciens strains to delete genes within the T-DNA region from the Ti plasmid and insert genes of interest. Selectable marker genes, like those for antibiotic or herbicide resistance, are often used alongside genes for agronomic traits, like fungal disease resistance (Figure 2) [71, 72]. Explants were co-cultured with A. tumefaciens strain containing the neomycin phosphotransferase (nptII) genes. GUS histochemical analysis using the pBIN19 plasmid showed the vigor hypocotyl able to provide regeneration efficiency compared to cotyledons. Antibiotic phytotoxicity negatively affected explant regenerative ability during Agrobacterium co-culture, reducing plant regeneration rates for both hypocotyl and cotyledon of C. sativa. Cannabis seedlings showed less susceptibility to transformation by A. tumefaciens due to the influence of variety on susceptibility to Agrobacterium transformation treated with 1% H2O2 for seed sterilisation and germination [73].
Cutting leaf sections may induce secondary metabolite production, inhibiting A. tumefaciens growth and reducing TF (Transfer frequency). Cannabis extracts inhibited the development of 18 out of the 19 tested microbes. The inhibition of Agrobacterium growth by C. sativa metabolites must be confirmed and properly analysed to determine its impact on different Cannabis genotypes. [74]. Non-expression of the pathogenesis-related protein 1 (NPR1) gene plays a crucial role in plants' salicylic acid-dependent pathway of systemic acquired resistance (SAR). Transformation with NPR1 or bar genes in Agrobacterium Strain EHA105 showed higher Transformation Frequency (TF) than strain GV3101. Cannabis genotypes likely influence transformation frequencies through their response to callus development [74]. Excising BBM and WUS from transgenic plants is crucial because some adverse effects from these genes limit their application, although these two factors are significant for regeneration. Additional developmental genes like SHOOT MERISTEMLESS (STM) and ISOPENTENYL TRANSFERASE (IPT) induced shoot organogenesis. The GROWTH-REGULATING FACTOR (GRF) and GRF-INTERACTING FACTOR (GIF) transcriptional complex boosted genetic transformation in Cannabis sativa, proved through CRISPR/Cas9 technology [75]. The susceptibility of genotypes to Agrobacterium has sparked interest in Agrobacterium-mediated gene transformation in Cannabis. However, challenges like low transformation efficiency, regeneration rates, chimeric regeneration, and transgene inactivation have been reported. To address these obstacles, it is essential to investigate factors like Agrobacterium strains, explants infection treatments, selection markers, chimerism eliminating, promoters, and translational enhancers [15].
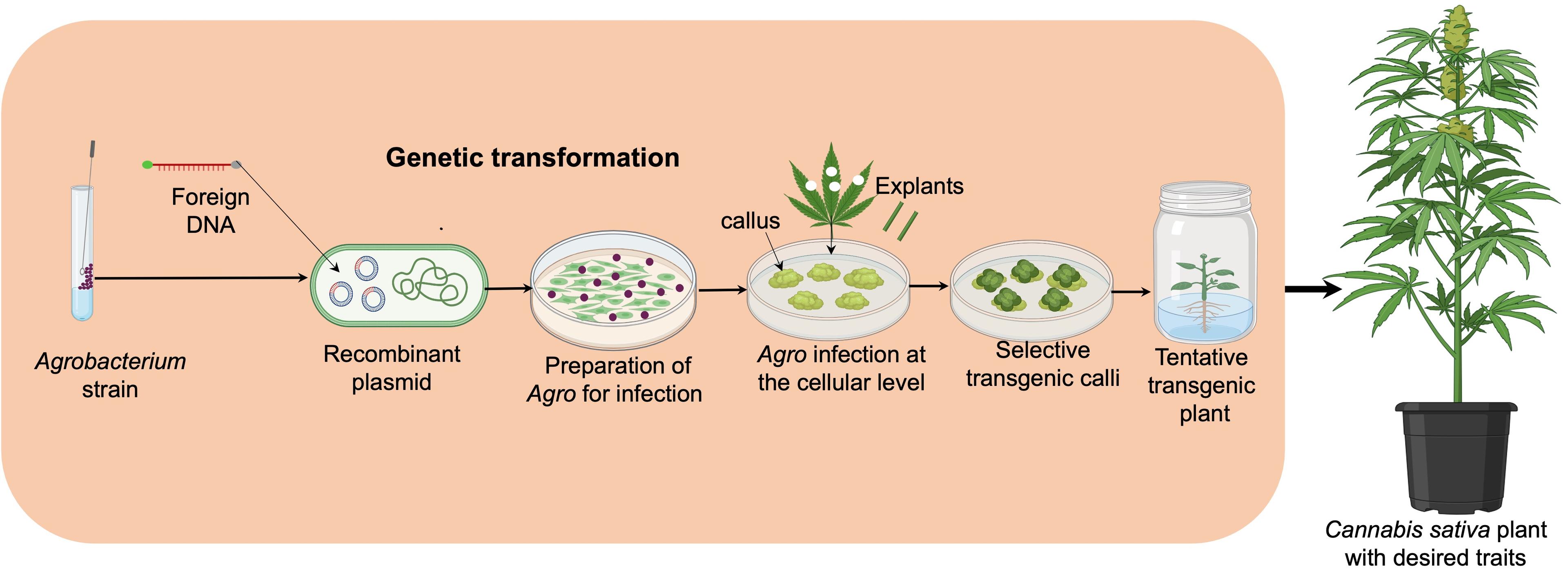
Table 1. Genetic manipulations in Cannabis sativa L.
Hairy root culture of Cannabis sativa
Limited information on the constituents and biological activities of hemp roots is available in the literature. Studies have shown that aqueous extract of the roots of C. sativa has anti-inflammatory and anti-asthmatic properties due to its unique active compounds. Hairy roots, which grow faster and produce valuable metabolites, can be induced with various agents to enhance the production of bioactive compounds [81]. Agrobacterium rhizogenes, is a Gram-negative soil bacterium that creates hairy root syndromes as an output of hormonal changes. The infection begins with the bacterium moving towards wounded plant sites, attracted by simple phenolic compounds. A. rhizogenes then invades the plant tissue and transfers T-DNA from its Ri-plasmid using virulence (vir) genes. This T-DNA integrates into the plant genome, leading to hairy root development and opine synthesis for bacterial nutrition. Essential genes, such as root-inducing “rol” genes (rolA, rolB, rolC, and rolD), play a crucial role in modulating plant processes like phytohormone synthesis and metabolism. Among these, rolB is a significant regulator of secondary metabolism, activating specific transcription factors for metabolic pathways (Figure 3) [81, 82]. Triterpenoids have been identified in hemp roots, showing bioactive effects—the potential enhancement of triterpenoid accumulation in the roots of C. sativa through elicitation. Hairy roots were established successfully, exhibiting 2.02-fold higher triterpenoid content than natural roots. Elicited hairy roots with 75 μM salicylic acid showed 1.95-fold higher friedelin and epifriedelanol levels than untreated hairy roots. This suggested that the optimising elicitation of hairy root cultures could be a viable method for enhancing triterpenoid production. [81, 82].Very few strains are tested for Hairy root culture-based Secondary metabolites production in Cannabis sativa. Future research should be focused on multi-strain trials available in Databases.
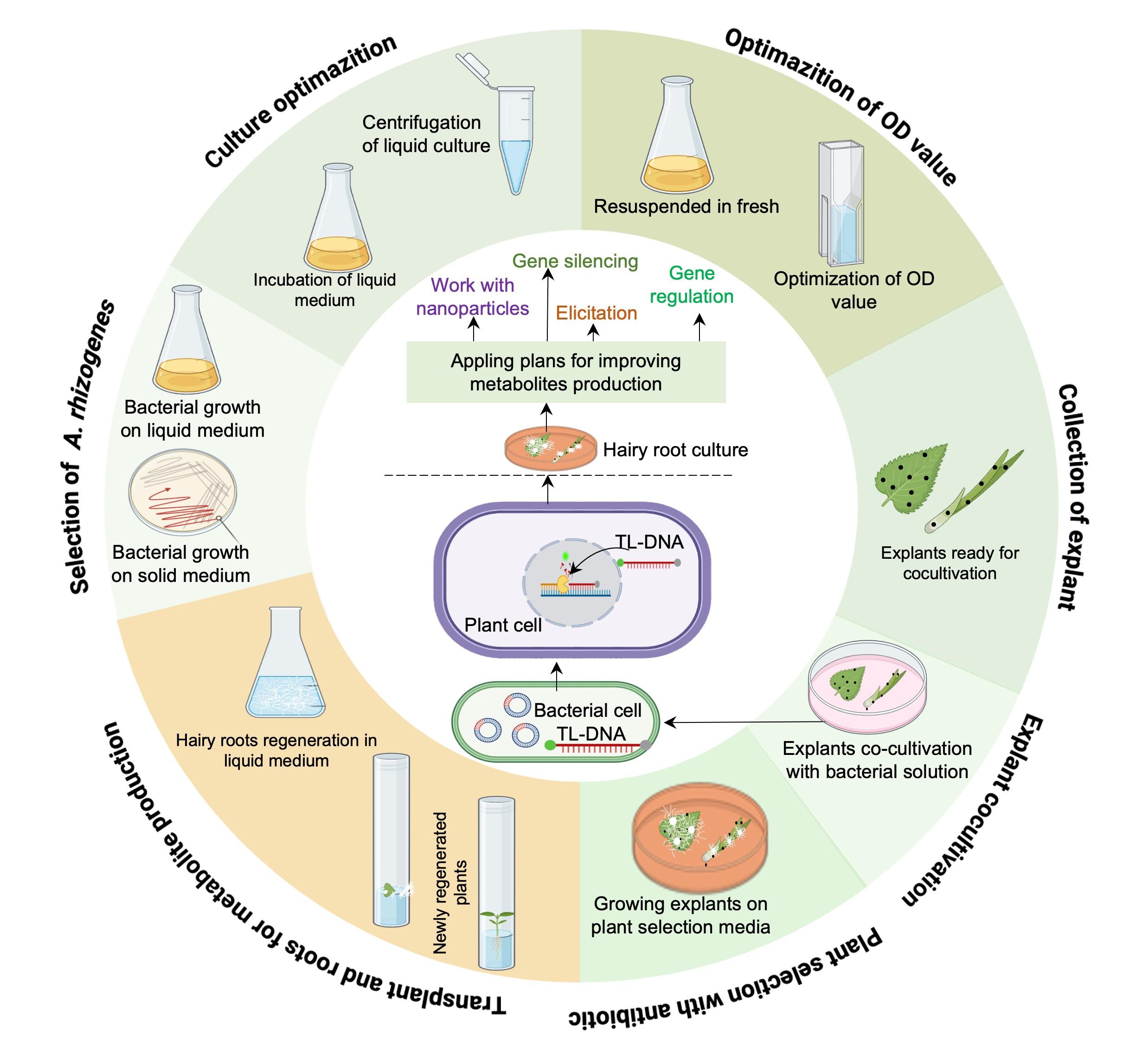
ELICITATION IN CANNABIS SATIVA
Plants produce secondary metabolites to adapt to various stresses, which have industrial applications and potential for commercialisation. Plant cell/tissue culture is an alternative method for in vitro production of these metabolites, with elicitation being an essential technique. Different elicitors (e.g. abiotic or biotic) induce secondary metabolites, osmolytes, antioxidant enzymes as a response of plant defence mechanisms [83-87]. However, the integrating transcriptomics, proteomics, and metabolomics with system biology can help discover new genes and pathways for metabolic engineering to increase secondary metabolite yield [88].
Elicitors interact with specific receptors on the plasma membrane, depolarizing and activating channels like K+/H+ antiporter, causing cytoplasmic acidification and signalling for secondary metabolite production. Receptor perception can also trigger G-protein-linked receptors or the mitogen-activated protein kinase (MAPK) cascade, resulting in Ca2+ fluxes and activation of intracellular processes. G-protein-linked receptors activate phospholipases C (PLC), phosphatidylinositol-4, and 5-bisphosphate (PIP2), leading to the production of secondary messengers like diacylglycerol (DAG) and inositol-1, 4,5-trisphosphate (IP3), which activates protein kinase C (PKC) and mobilizes Ca2+ ions. This process can generate reactive oxygen species (ROS) and regulate the expression of defence genes. Crosstalk between signalling pathways, such as jasmonic acid (JA) and ethylene (ET), can regulate gene expression against various es (Figure 4) [88, 89].
In a study, CHT, SA, JA, and MeJA were tested, along with elicitation and precursor feeding during the exponential growth phase of cell suspensions. Secondary metabolites are primarily synthesised after cell growth enters the exponential phase, as plant cells need to adjust to the new environment during the lag phase. JA and MeJA treatments increase secondary metabolite accumulation but can inhibit growth by suppressing mitosis. Precursor feeding and elicitor treatments can enhance secondary metabolite synthesis by providing substrate biosynthesis pathways. A beneficial strategy involved MeJA and tyrosine (Tyr) precursor feeding, which increased the activity and expression of PAL and tyrosine aminotransferase (TAT). 1H-NMR analyses identified aromatic compounds, including 4-hydroxyphenylpyruvate (4-HPP), tyrosol, and 2,2-diphenyl-1-picrylhydrazyl (DPPH) [89]. Recent developments in the application of elicitors in coordinating antioxidant defence in various medicinal and other plants [90]. However, elicitor responsive plant defence it is crucial for producing plant metabolites in Cannabis and other plant species.
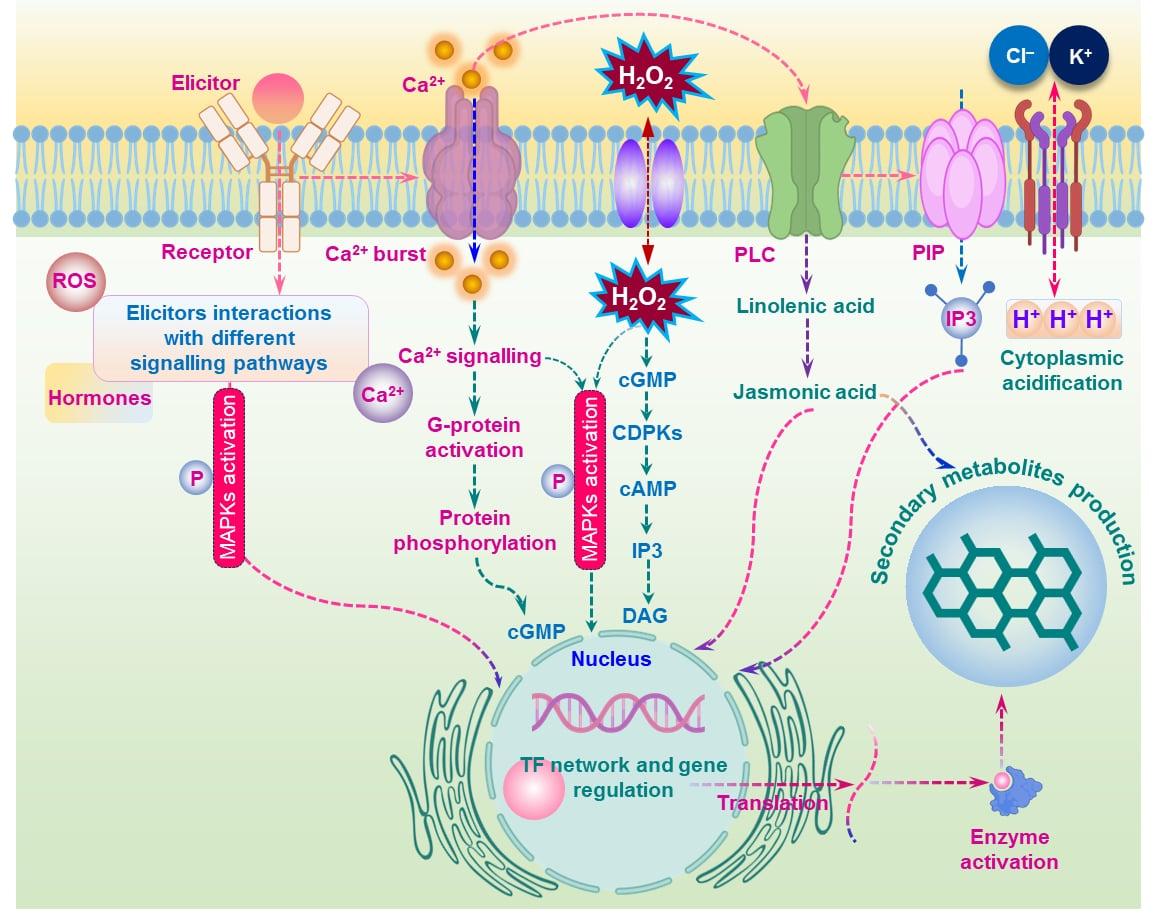
CONCLUSION AND FUTURE DIRECTIONS
This study provides an extensive overview of plant improvement and metabolite production in Cannabis through various biotechnological tools. Regarding its pharmaceutical and medicinal benefits, the plant has been improved for metabolite-containing traits using numerous biotechnological techniques. Significant advancements have been achieved through in vitro plant tissue culture, protoplast culture, genetic transformation, and modern gene editing approaches. These developments have greatly improved plant development, particularly enhanced growth with desired traits. The phytochemicals and bioactive compounds produced by Cannabis have significantly enriched its pharmaceutical and medicinal value globally. Despite these biotechnological and pharmaceutical advancements in Cannabis, some limitations still exist, which should be addressed and solved. However, several factors, especially the lack of robust genotype protocols, still show difficulties. Therefore, the growth and development of regulatory genes need to be studied with greater precision to produce transgenic Cannabis and enhance in vitro cell culture-based secondary metabolite production on an industrial scale. Furthermore, it is crucial to separate toxic or harmful metabolites, identify the factors involved in metabolite networks, and isolate novel genes encoding proteins or enzymes related to effective metabolite production in Cannabis. The modern CRISPR/Cas gene editing approach offers a potential solution to address these challenges in C. sativa.
ACKNOWLEDGEMENTS
This work was supported by the Promotion (NO: P0023710) of Innovative Businesses for Regulation-Free Special Zones funded by the Ministry of SMEs and Startups (MSS, Korea).
AUTHOR CONTRIBUTIONS
SMA and HWC conceived the research plan and wrote the initial draft of the manuscript. MIUH, SS, and MMR wrote and edited the manuscript. AK supported to figure drawing. All the authors approved the final version of the manuscript.
CONFLICTS OF INTEREST
There is no conflict of interest among the authors.
References
- [1]McPartland JM, Hegman W, Long T. Cannabis in asia: Its center of origin and early cultivation, based on a synthesis of subfossil pollen and archaeobotanical studies. Vegetation History and Archaeobotany. 2019;28:691-702.
- [2]Sharma D, Bhushan S, et al. Cannabis as a potent therapeutic agent for pharmaceutical drugs: Recent advancement in drug discovery and human healthcare. In: Agrawal DC, Kumar R, Dhanasekaran M, editors. Cannabis/marijuana for healthcare. Singapore: Springer Nature Singapore; 2022. p. 77-99.
- [3]Hesami M, Pepe M, et al. New insight into ornamental applications of cannabis: Perspectives and challenges. Plants. 2022;11:2383.
- [4]Tanney CAS, Backer R, et al. Cannabis glandular trichomes: A cellular metabolite factory. Frontiers in Plant Science. 2021;12.
- [5]Hesami M, Pepe M, et al. Recent advances in cannabis biotechnology. Industrial Crops and Products. 2020;158:113026.
- [6]Shahbazi F, Grandi V, et al. Cannabinoids and cannabinoid receptors: The story so far. iScience. 2020;23:101301.
- [7]Zou S, Kumar U. Cannabinoid receptors and the endocannabinoid system: Signaling and function in the central nervous system. International Journal of Molecular Sciences. 2018;19:833.
- [8]Patel M, Finlay DB, Glass M. Biased agonism at the cannabinoid receptors – evidence from synthetic cannabinoid receptor agonists. Cellular Signalling. 2021;78:109865.
- [9]Magham SV, Thaggikuppe krishnamurthy P, et al. Cannabinoid receptor 2 selective agonists and alzheimer's disease: An insight into the therapeutic potentials. Journal of Neuroscience Research. 2021;99:2888-905.
- [10]Kovalchuk A, Wang B, et al. Fighting the storm: Could novel anti-tnfα and anti-il-6 c. Sativa cultivars tame cytokine storm in covid-19? Aging (Albany NY). 2021;13:1571-90.
- [11]Nguyen LC, Yang D, et al. Cannabidiol inhibits sars-cov-2 replication through induction of the host er stress and innate immune responses. Science Advances. 2022;8:eabi6110.
- [12]Onaivi ES, Sharma V. Cannabis for covid-19: Can cannabinoids quell the cytokine storm? Future Science OA. 2020;6.
- [13]Andre CM, Hausman J-F, Guerriero G. Cannabis sativa: The plant of the thousand and one molecules. Frontiers in Plant Science. 2016;7.
- [14]Adhikary D, Kulkarni M, et al. Medical cannabis and industrial hemp tissue culture: Present status and future potential. Frontiers in Plant Science. 2021;12.
- [15]Hesami M, Baiton A, et al. Advances and perspectives in tissue culture and genetic engineering of cannabis. International Journal of Molecular Sciences. 2021;22:5671.
- [16]Laverty KU, Stout JM, et al. A physical and genetic map of Cannabis sativa identifies extensive rearrangements at the thc/cbd acid synthase loci. Genome Research. 2019;29:146-56.
- [17]Thomas F, Schmidt C, Kayser O. Bioengineering studies and pathway modeling of the heterologous biosynthesis of tetrahydrocannabinolic acid in yeast. Applied Microbiology and Biotechnology. 2020;104:9551-63.
- [18]Bhajan S, Hasan M, et al. An efficient approach of in vitro plant regeneration and propagation o f mungbean [Vigna radiata (L.) wilczek]. Plant Trends.2(3):46-56.
- [19]Niazian M. Application of genetics and biotechnology for improving medicinal plants. Planta. 2019;249:953-73.
- [20]Monthony AS, Kyne ST, et al. Recalcitrance of Cannabis sativa to de novo regeneration; a multi-genotype replication study. PLOS ONE. 2021;16:e0235525.
- [21]Wróbel T, Dreger M, et al. The application of plant in vitro cultures in cannabinoid production. Biotechnology Letters. 2018;40:445-54.
- [22]Barcaccia G, Palumbo F, et al. Potentials and challenges of genomics for breeding cannabis cultivars. Frontiers in Plant Science. 2020;11.
- [23]Page SRG, Monthony AS, Jones AMP. Dkw basal salts improve micropropagation and callogenesis compared to ms basal salts in multiple commercial cultivars of Cannabis sativa. bioRxiv. 2020:2020.02.07.939181.
- [24]Wróbel T, Dreger M, et al. Modified nodal cuttings and shoot tips protocol for rapid regeneration of cannabis sativa l. Journal of Natural Fibers. 2022;19:536-45.
- [25]Beard KM, Boling AWH, Bargmann BOR. Protoplast isolation, transient transformation, and flow-cytometric analysis of reporter-gene activation in cannabis sativa l. Industrial Crops and Products. 2021;164:113360.
- [26]Monthony AS, Page SR, et al. The past, present and future of Cannabis sativa tissue culture. Plants. 2021;10:185.
- [27]Halstead MA, Garfinkel AR, et al. Hemp growth in vitro and in vivo: A comparison of growing media and growing environments across 10 accessions. Hort Science. 2022;57:1041-7.
- [28]Cassells AC. Pathogen and biological contamination management in plant tissue culture: Phytopathogens, vitro pathogens, and vitro pests. In: Loyola-Vargas VM, Ochoa-Alejo N, editors. Plant cell culture protocols. Totowa, NJ: Humana Press; 2012. p. 57-80.
- [29]Weston RJ. The contribution of catalase and other natural products to the antibacterial activity of honey: A review. Food Chemistry. 2000;71:235-9.
- [30]Wojtyla Ł, Lechowska K, et al. Different modes of hydrogen peroxide action during seed germination. Frontiers in Plant Science. 2016;7.
- [31]Hesami M, Pepe M, et al. Modeling and optimizing in vitro seed germination of industrial hemp (cannabis sativa l.). Industrial Crops and Products. 2021;170:113753.
- [32]Ahsan SM, Shin JH, Choi HW. Availability of hydrogen peroxide solutions as a germination liquid medium for contamination-free in vitro seedling development of Cannabis sativa. Horticultural Science and Technology. 2022;40:605-13.
- [33]Pepe M, Hesami M, Jones AMP. Machine learning-mediated development and optimization of disinfection protocol and scarification method for improved in vitro germination of cannabis seeds. Plants. 2021;10:2397.
- [34]Galán-Ávila A, García-Fortea E, et al. Development of a direct in vitro plant regeneration protocol from cannabis sativa l. Seedling explants: Developmental morphology of shoot regeneration and ploidy level of regenerated plants. Frontiers in Plant Science. 2020;11.
- [35]Hesami M, Adamek K, et al. Effect of explant source on phenotypic changes of in vitro grown cannabis plantlets over multiple subcultures. Biology. 2023;12:443.
- [36]Murphy R, Adelberg J. Physical factors increased quantity and quality of micropropagated shoots of Cannabis sativa L. In a repeated harvest system with ex vitro rooting. In Vitro Cellular & Developmental Biology - Plant. 2021;57:923-31.
- [37]Zayed EMM, Zein El Din AFM, et al. Floral reversion of mature inflorescence of date palm in vitro. Annals of Agricultural Sciences. 2016;61:125-33.
- [38]Monthony AS, Bagheri S, et al. Flower power: Floral reversion as a viable alternative to nodal micropropagation in cannabis sativa. In Vitro Cellular & Developmental Biology - Plant. 2021;57:1018-30.
- [39]Holmes JE, Lung S, et al. Variables affecting shoot growth and plantlet recovery in tissue cultures of drug-type Cannabis sativa L. Frontiers in Plant Science. 2021;12.
- [40]Smýkalová I, Vrbová M, et al. The effects of novel synthetic cytokinin derivatives and endogenous cytokinins on the in vitro growth responses of hemp (Cannabis sativa L.) explants. Plant Cell, Tissue and Organ Culture (PCTOC). 2019;139:381-94.
- [41]Barbier FF, Dun EA, Beveridge CA. Apical dominance. Current Biology. 2017;27:R864-R5.
- [42]Kebrom TH. A growing stem inhibits bud outgrowth – the overlooked theory of apical dominance. Frontiers in Plant Science. 2017;8.
- [43]Bertheloot J, Barbier F, et al. Sugar availability suppresses the auxin-induced strigolactone pathway to promote bud outgrowth. New Phytologist. 2020;225:866-79.
- [44]Prusinkiewicz P, Crawford S, et al. Control of bud activation by an auxin transport switch. Proceedings of the National Academy of Sciences. 2009;106:17431-6.
- [45]Cheng ZJ, Wang L, et al. Pattern of auxin and cytokinin responses for shoot meristem induction results from the regulation of cytokinin biosynthesis by auxin response factor3 Plant Physiology. 2012;161:240-51.
- [46]Luisi A, Lorenzi R, Sorce C. Strigolactone may interact with gibberellin to control apical dominance in pea (Pisum sativum). Plant Growth Regulation. 2011;65:415-9.
- [47]Li M, Wei Q, et al. The effect of auxin and strigolactone on atp/adp isopentenyltransferase expression and the regulation of apical dominance in peach. Plant Cell Reports. 2018;37:1693-705.
- [48]Müller D, Waldie T, et al. Cytokinin is required for escape but not release from auxin mediated apical dominance. The Plant Journal. 2015;82:874-86.
- [49]Dreger M, Szalata M. The effect of tiba and npa on shoot regeneration of Cannabis sativa L. Epicotyl explants. Agronomy. 2022;12:104.
- [50]Šenkyřík JB, Křivánková T, et al. Investigation of the effect of the auxin antagonist peo-iaa on cannabinoid gene expression and content in Cannabis sativa L. Plants under in vitro conditions. Plants. 2023;12:1664.
- [51]Žukauskaitė A, Saiz-Fernández I, et al. New peo-iaa-inspired anti-auxins: Synthesis, biological activity, and possible application in hemp (Cannabis sativa L.) micropropagation. Journal of Plant Growth Regulation. 2023;42:7547-63.
- [52]Lumsden, P.J., Vince-Prue, D. The perception of dusk signals in photoperiodic time-measurement. Physiol Plant. 1984;60:427-32.
- [53]Moher M, Jones M, Zheng Y. Photoperiodic response of in vitro cannabis sativa plants. HortScience horts. 2021;56:108-13.
- [54]Ioannidis K, Tomprou I, et al. Genetic evaluation of in vitro micropropagated and regenerated plants of cannabis sativa l. Using ssr molecular markers. Plants. 2022;11:2569.
- [55]Patel P, Sarswat SK, Modi A. Chapter 12 - strategies to overcome explant recalcitrance under in vitro conditions. In: Chandra Rai A, Kumar A, et al., editors. Advances in plant tissue culture: Academic Press; 2022. p. 283-94.
- [56]Hesami M, Pepe M, et al. Transcriptomic profiling of embryogenic and non-embryogenic callus provides new insight into the nature of recalcitrance in cannabis. International Journal of Molecular Sciences. 2023;24:14625.
- [57]Murthy HN, Joseph KS, et al. Bioreactor systems for micropropagation of plants: Present scenario and future prospects. Frontiers in Plant Science. 2023;14.
- [58]Mirzabe AH, Hajiahmad A, et al. Temporary immersion systems (tiss): A comprehensive review. Journal of Biotechnology. 2022;357:56-83.
- [59]Rico S, Garrido J, et al. A temporary immersion system to improve cannabis sativa micropropagation. Frontiers in Plant Science. 2022;13.
- [60]Parsons JL, Martin SL, et al. Polyploidization for the genetic improvement of Cannabis sativa. Frontiers in Plant Science. 2019;10.
- [61]Gantait S, Mukherjee E. Induced autopolyploidy—a promising approach for enhanced biosynthesis of plant secondary metabolites: An insight. Journal of Genetic Engineering and Biotechnology. 2021;19:4.
- [62]Madani H, Escrich A, et al. Effect of polyploidy induction on natural metabolite production in medicinal plants. Biomolecules. 2021;11:899.
- [63]Bharati R, Fernández-Cusimamani E, et al. Oryzalin induces polyploids with superior morphology and increased levels of essential oil production in Mentha spicata L. Industrial Crops and Products. 2023;198:116683.
- [64]Naing AH, Adedeji OS, Kim CK. Protoplast technology in ornamental plants: Current progress and potential applications on genetic improvement. Scientia Horticulturae. 2021;283:110043.
- [65]Yue J-J, Yuan J-L, et al. Protoplasts: From isolation to crispr/cas genome editing application. Frontiers in Genome Editing. 2021;3.
- [66]Kim AL, Yun YJ, et al. Establishment of efficient cannabis (Cannabis sativa L.) protoplast isolation and transient expression condition. Plant Biotechnology Reports. 2022;16:613-9.
- [67]Matchett-Oates L, Mohamaden E, et al. Development of a robust transient expression screening system in protoplasts of cannabis. In Vitro Cellular & Developmental Biology - Plant. 2021;57:1040-50.
- [68]Brandt KM, Gunn H, et al. A streamlined protocol for wheat (Triticum aestivum) protoplast isolation and transformation with crispr-cas ribonucleoprotein complexes. Frontiers in Plant Science. 2020;11.
- [69]Tabatabaei I, Dal Bosco C, et al. A highly efficient sulfadiazine selection system for the generation of transgenic plants and algae. Plant Biotechnology Journal. 2019;17:638-49.
- [70]Islam MS, Akter M, et al. Isolation of agrobacterium tumefaciens strains from crown gall sample of dicot plants in Bangladesh. Current Research in Bacteriology. 2010;3:27-36.
- [71]Wally O, Jayaraj J, Punja ZK. Broad-spectrum disease resistance to necrotrophic and biotrophic pathogens in transgenic carrots (Daucus carota L.) expressing an arabidopsis npr1 gene. Planta. 2009;231:131-41.
- [72]Silva KJP, Mahna N, et al. Npr1 as a transgenic crop protection strategy in horticultural species. Horticulture Research. 2018;5.
- [73]Sorokin A, Yadav NS, et al. Transient expression of the β-glucuronidase gene in Cannabis sativa varieties. Plant Signaling & Behavior. 2020;15:1780037.
- [74]Holmes JE, Punja ZK. Agrobacterium-mediated transformation of thc-containing Cannabis sativa L. Yields a high frequency of transgenic calli expressing bialaphos resistance and non-expressor of pr1 (npr1) genes. Botany. 2023;101:498-512.
- [75]Zhang X, Xu G, et al. Establishment of an agrobacterium-mediated genetic transformation and crispr/cas9-mediated targeted mutagenesis in hemp (Cannabis sativa L.). Plant Biotechnology Journal. 2021;19:1979-87.
- [76]Galán-Ávila A, Gramazio P, et al. A novel and rapid method for agrobacterium-mediated production of stably transformed cannabis sativa l. Plants. Industrial Crops and Products. 2021;170:113691.
- [77]Zhang X, Xu G, et al. Establishment of an agrobacterium-mediated genetic transformation and crispr/cas9-mediated targeted mutagenesis in hemp (Cannabis sativa L.). Plant Biotechnol J. 2021;19:1979-87.
- [78]Matchett-Oates L, Mohamaden E, et al. Development of a robust transient expression screening system in protoplasts of cannabis. In Vitro Cellular & Developmental Biology - Plant. 2021;57:1040 - 50.
- [79]Beard KM, Boling AWH, Bargmann BOR. Protoplast isolation, transient transformation, and flow-cytometric analysis of reporter-gene activation in Cannabis sativa L. Industrial Crops and Products. 2021.
- [80]Sorokin A, Yadav NS, et al. Transient expression of the β-glucuronidase gene in Cannabis sativa varieties. Plant Signaling & Behavior. 2020;15.
- [81]Biswas D, Chakraborty A, et al. Hairy root culture: A potent method for improved secondary metabolite production of solanaceous plants. Frontiers in Plant Science. 2023;14. 1197555.
- [82]Kobtrakul K, Rani D, et al. Elicitation enhances the production of friedelin and epifriedelanol in hairy root cultures of cannabis sativa l. Frontiers in Plant Science. 2023;14.
- [83]Chauhan J, Srivastava JP, et al. Alterations of oxidative stress indicators, antioxidant enzymes, soluble sugars, and amino acids in mustard [Brassica juncea (L.) Czern and coss.] in response to varying sowing time, and field temperature. Frontiers in Plant Science. 2022;13. 875009.
- [84]Raza A, Bhardwaj S, et al. Trehalose: A sugar molecule involved in temperature stress management in plants. The Crop Journal. 2024;12:1-16.
- [85]Singh D, Sharma NL, et al. Exogenous hydrogen sulfide alleviates chromium toxicity by modulating chromium, nutrients and reactive oxygen species accumulation, and antioxidant defence system in mungbean (Vigna radiata L.) seedlings. Plant Physiology and Biochemistry. 2023;200:107767.
- [86]Akash S, Etha S, et al. In vitro evaluation of antioxidant and antimicrobial properties of car dia mocleodii leaf extract. Plant Trends.2:38.
- [87]Mahora M, Kigundu A, Waihenya R. Screening of phytochemicals, antioxidant activity, and in vivo safety profile of the hydroethanolic peel extract of musa sapientum. Plant Trends. 2(1):1-15.
- [88]Narayani M, Srivastava S. Elicitation: A stimulation of stress in in vitro plant cell/tissue cultures for enhancement of secondary metabolite production. Phytochemistry Reviews. 2017;16:1227-52.
- [89]Gabotti D, Locatelli F, et al. Cell suspensions of Cannabis sativa (var. Futura): Effect of elicitation on metabolite content and antioxidant activity. Molecules. 2019;24:4056.
- [90]Hoque M, Jony M, et al. Coordination of elicitors and ascorbate-glutathione cycle: A vital nex us for mitigating post-harvest injury and losses of cultivated fruits. Plant Trends.2(2):24-37.